This article was first published on Clinical Lab Products.
An Overview of Lyme Disease
Lyme disease is an emerging tick-borne infection caused by members of the Borrelia burgdorferi species complex of bacteria, first characterized in 1975 in Old Lyme, Connecticut. The hallmark of Lyme disease is a circular, expanding rash known as erythema migrans, as well as other non-specific, flu-like symptoms. Initial infection of the skin typically resolves with antibiotic treatment; however, if mis-diagnosed or untreated, infecting Borrelia spp. can disseminate to distal sites through the bloodstream and lymphatic system, leading to chronic infection [1]. Chronic Lyme disease can manifest as a broad range of highly variable long-term sequelae, including neurological complications (neuroborreliosis), meningitis, heart problems, and Lyme arthritis [1].
The Borrelia genus of spirochetes (spiral-shaped bacteria) are characterized by their periplasmic endoflagella, which they use to propel themselves in a corkscrew-like motion (figure 1). The Borrelia burgdorferi species complex in its broadest sense (sensu lato) comprises at least 20 distinct genospecies [2], of which four are thought to be the primary causative agents of Lyme disease: in America, B. burgdorferi sensu stricto (in the strict sense) and the newly identified B. mayonii; in Europe and Asia, B. afzelii and B. garinii. Each pathogenic species is associated with different enzootic lifecycles and clinical manifestations of infection. B. afzelii, for example, is mostly associated with manifestations of the skin, whereas B. garinii is considered to be the most neurotropic, and B. burgdorferi sensu stricto the most arthritogenic.
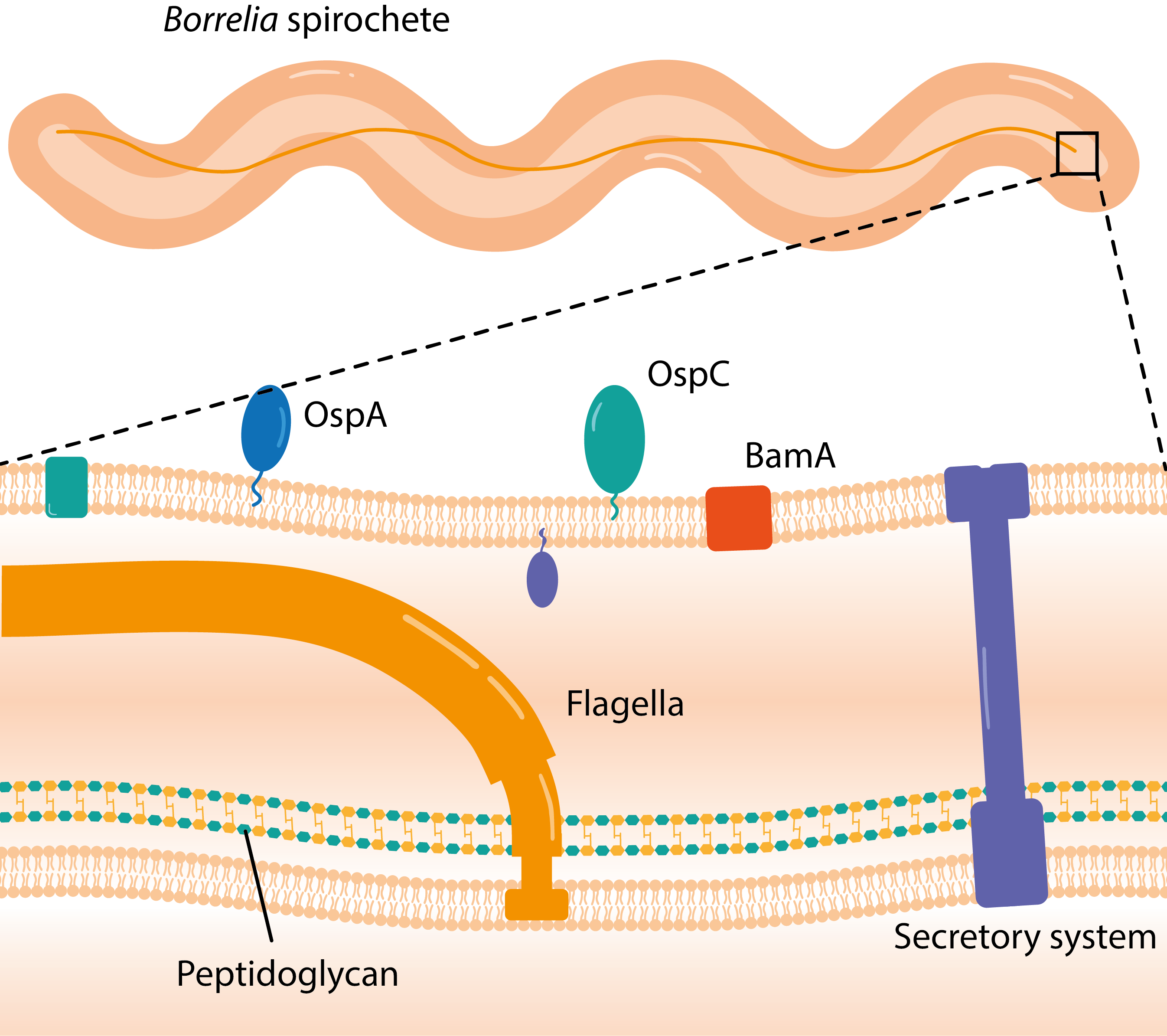
Figure 1. Diagram showing the membrane structure of Borrelia, with periplasm and major membrane components labelled.
Borrelia spp. are transmitted by hard-bodied ticks of the Ixodes genus, which carry other notable pathogens, including Tick-Borne Encephalitis virus (TBEV), Powassan virus, Babesia and Anaplasma. Ixodid ticks are just a few millimeters in diameter and feed on a diverse range of hosts found in grassy, wooded areas, including small rodents and birds, and larger mammals, such as wild boar, deer and cattle. Ticks, such as I. scapularis, and I. pacificus in North America, and I. ricinus in Europe, acquire Borrelia from reservoirs during blood meals and transmit the spirochete to new hosts during subsequent feeds. These ticks have three blood-feeding stages — larva, nymph and adult — which take 2–3 years to pass through. To develop to the next stage, or lay eggs in the case of adults, a single blood meal is needed, typically taking 36–48 hours. The majority of Borrelia infections are caused by tick nymphs, which are significantly smaller than adults, and are abundant during the spring-summer months when human and animal activity peaks [3].
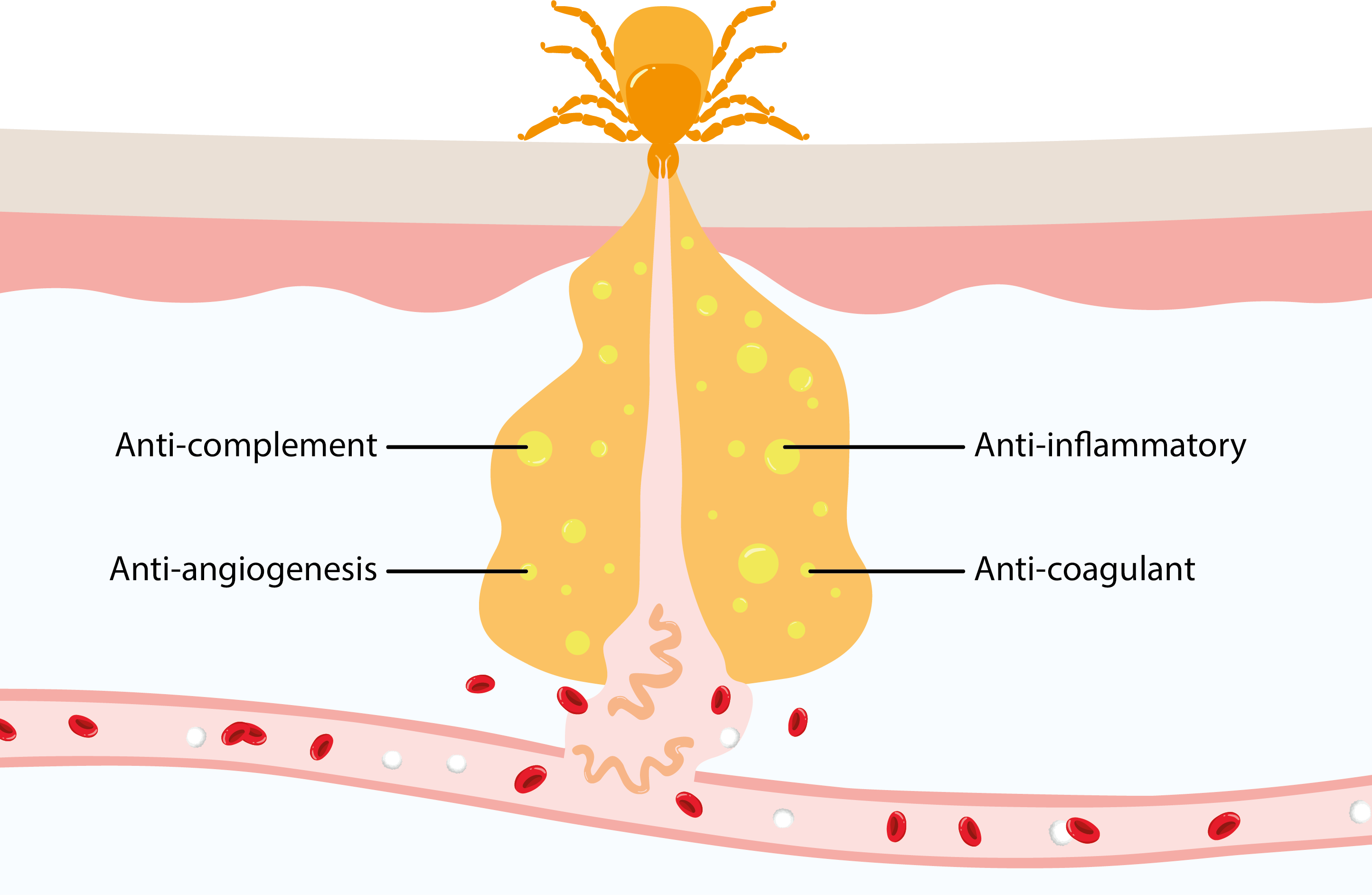
Figure 2. Diagram showing the release of salivary immune evasion factors and Borrelia spirochetes by feeding tick. Adapted from Kurokawa et al. [4].
To facilitate feeding, Ixodid ticks secrete a saliva that glues themselves to their host (hence Ixodes, from the Greek word ixos meaning “glue”). Once attached, Ixodid ticks lacerate the vasculature of the host’s dermis and draw blood from the lesion. However, this destructive style of feeding risks triggering host defense mechanisms, which has led ticks to develop an array of pharmacologically active molecules that prevent hemostasis, inflammation, immunity, and wound healing (figure 2). Like their tick vectors, Borrelia spp. also have a vested interest in evading host immune responses, employing tactics such as downregulation of their outer surface proteins (OspA-C), switching of gene expression loci, and neutralization of host complement systems [4]. In combination, these immunosuppressive activities facilitate dissemination of Borrelia and the multi-organ forms of disease characterized in chronic infections.
Since its discovery, Lyme disease has become the most common and fastest growing vector-borne illness in the Western world. There are estimated to be approximately 600,000 new cases in the United States and Europe each year [5, 6], with 1–3 million people thought to be currently suffering from the long-term effects of Lyme disease in the United States alone [7]. Though a rapidly emerging disease, Borrelia has likely existed as a human pathogen for millennia, evidenced by genome sequencing of a 5,000-year-old Tyrolean “iceman” in 2011, which found Borrelia DNA with 60% homology to the B. burgdorferi genome, consistent with an ancient infection [8]. The reasons for the emergence of Lyme disease in recent years is not clear. However, reforestation of farm land and ever-increasing suburban areas have created open spaces that are supporting larger host populations [9], which in combination with warming climates, are expected to continue accelerating the emergence of tick-borne diseases in the coming decades [3].
Currently, there are no specific means of preventing Lyme disease beyond general measures, such as prophylactic antibiotics, repellents, insecticides and covering of exposed skin. A vaccine was developed and licensed in the United States in the 90s but was withdrawn due to poor sales, largely attributed to cost and safety concerns [10]. Considering recent advancements in science and a growing burden of disease, various second-generation candidates are now in clinical trials, though it will likely be some time before they are commercially available.
The Difficulties in Diagnosis
Following identification of Borrelia as the etiological agent of Lyme disease in the late 20th century, laboratory diagnosis soon became a priority. However, the complexities of Borrelia infection present several unique problems that have made it a challenging and sometimes counter-intuitive disease to diagnose. Lyme disease is diverse in its clinical presentation and in the absence of a distinctive erythema migrans rash can easily escape recognition. Furthermore, despite its multisystemic nature, Lyme bacteremia is highly transient after initial infection, making isolation for culture or microscopic examination unreliable [2]. This in turn, has made polymerase chain reaction (PCR) ineffective as a stand-alone diagnostic method given the transience of bacterial DNA in bodily fluids [11].
With few alternatives available, antibody tests were developed to indirectly measure the immune response to infection, though initial performance was poor and unreliable. Addressing this, a conference was held in 1994, in Dearborn, Michigan, which established a 2-tiered testing (2TT) paradigm (figure 3). 2TT is carried-out as follows: upon identification of an erythema migrans rash or suspected tick bite, antibodies from the blood are analyzed with a sensitive enzyme-linked immunosorbent assay (ELISA). If positive, a Western blot (WB) or high-specificity ELISA is then used to ensure a true-positive result [12].
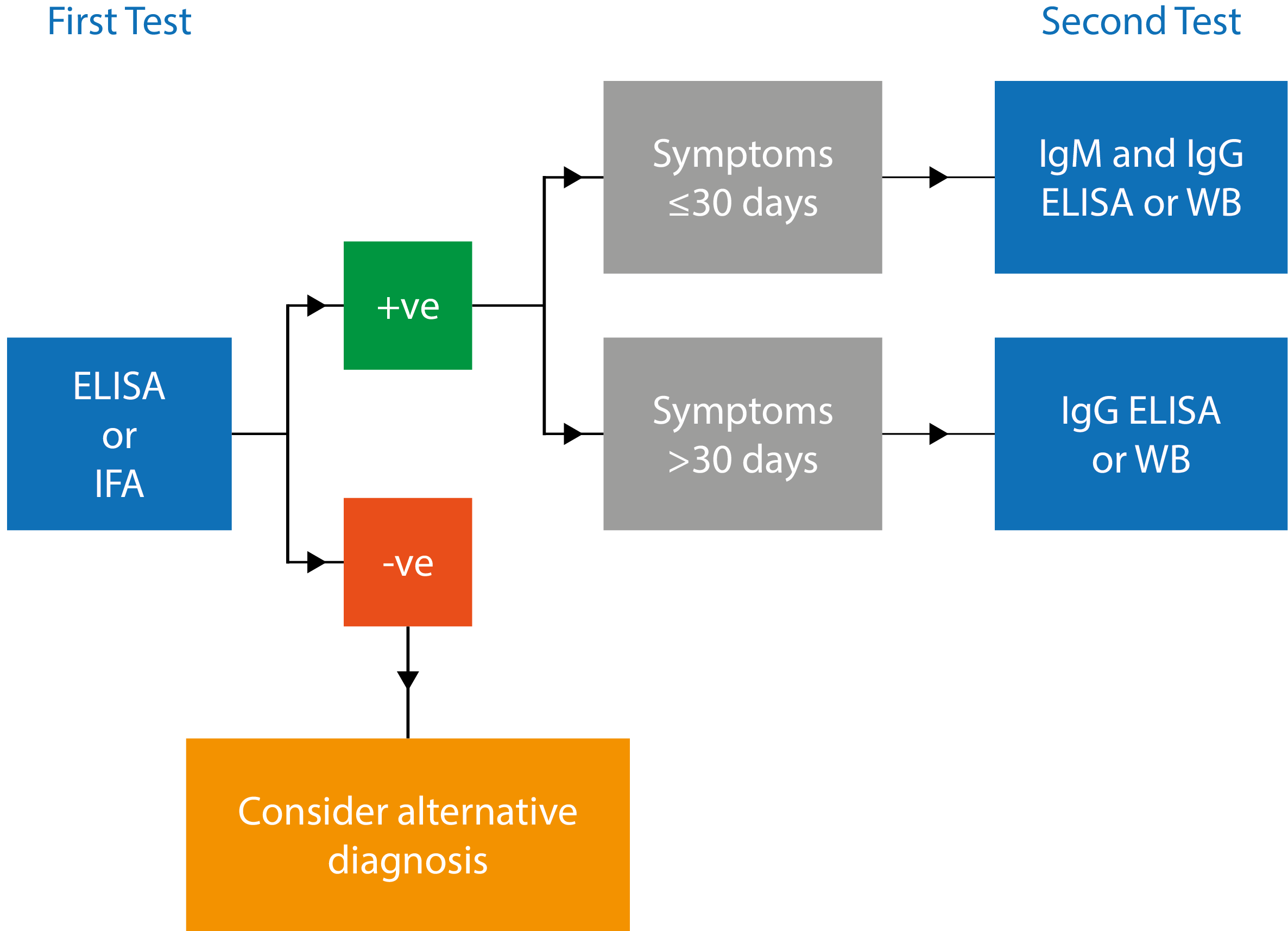
Figure 3. Schematic illustrating 2TT algorithm for diagnosis of Lyme disease.
Adapted from Centers for Disease Control and Prevention [13].
Over two decades later, 2TT remains the standard laboratory diagnosis for Lyme disease and continues to be recommended by leading public health agencies in both the United States and Europe. However, this paradigm suffers from well-recognized limitations. Firstly, although 2TT is generally sensitive and specific in late-stage disease, antibodies against Borrelia spp. are slow to develop, with IgM generally not being detectable for the first 1–2 weeks and IgG, 4–6 weeks after infection [2]. As a result, detection antibodies by ELISA is less than 40% sensitive during the first three weeks of infection when antibiotic treatment is most effective [14]. In addition, Borrelia spp. are highly pleomorphic over the course of a host infection cycle, with altered gene expression profiles that result in distinct immune responses [15]. This is further complicated by the antigenic heterogeneity of divergent Borrelia spp., and even heterologous strains of the same species that are easy to miss [2].
Other limitations of 2TT relate to its clinical utility and practicality. As recovered patients may be seropositive for many years post-infection, assays testing for IgG cannot reliably distinguish between active and historic infections, or reliably measure humoral responses to effective antibiotic treatment [16]. When combined with the fact that the majority of tests are performed in situations with low likelihood of disease, this creates an inherent risk of producing false-positive results which are both costly and time-consuming for healthcare providers and may draw attention away from the need to diagnose other serious diseases like multiple sclerosis or rheumatoid arthritis [17]. Finally, ELISAs and WBs require specialized equipment and training, and are constrained by long turnaround times (>24 h), high costs (>$400/test) and limited throughput [18]. This in particular limits the accessibility to Lyme disease testing, especially in populations far from clinical laboratories in rural and forested areas where tick bites are common.
Novel Serological Diagnostics
The accurate and timely diagnosis of Lyme disease is crucial, as delays, missed diagnosis, or inadequate treatment risk the development of more severe and chronic disease, with worsening prognosis [19]. Given the limitations of 2TT and the growing burden of Lyme disease, there has been a growing drive from the medical and public health communities to improve diagnosis.
The first major step in improving the serological diagnosis of Lyme disease was the development of novel antigenic targets. First generation ELISAs and WBs used whole cell Borrelia sonicates, which conferred sensitivity with a broad range of antigens, but allowed some antibodies to cross-react with unrelated bacterial epitopes, leading to false-positive results. Moreover, studies showed that many Borrelia antigens expressed in vivo during infection, were not being expressed under the non-native conditions of in vitro culture. To address this, large-scale antigen screens were carried out, leading to the discovery of a range of new targets, such as flagellin (FLA), decorin-binding protein (DbpA), outer surface protein C (OspC) and Vmp-like sequence E (VlsE) [20]. Recombinantly-expressed antigens and peptides (like VlsE’s C6) represented a significant improvement over whole cell sonicates, with specificities now typically exceeding 95% [21]. However, given the antigenic heterogeneity of Borrelia spp. and its variance over the time-course of infection, 2TT is still limited in its functionality.
Therefore, a novel generation of diagnostics are now being investigated, which can multiplex large panels of diverse antigenic targets. Joung and colleagues, for example, developed a multiplexed vertical flow assay (xVFA) that can detect a range of both early and late-stage antibodies using 13 immunospots (figure 4) [18]. In combination with a digital reader that employs a deep-learning algorithm, the test has shown to be twice as sensitive as 2TT for early-stage diagnosis and for a fraction of the cost.
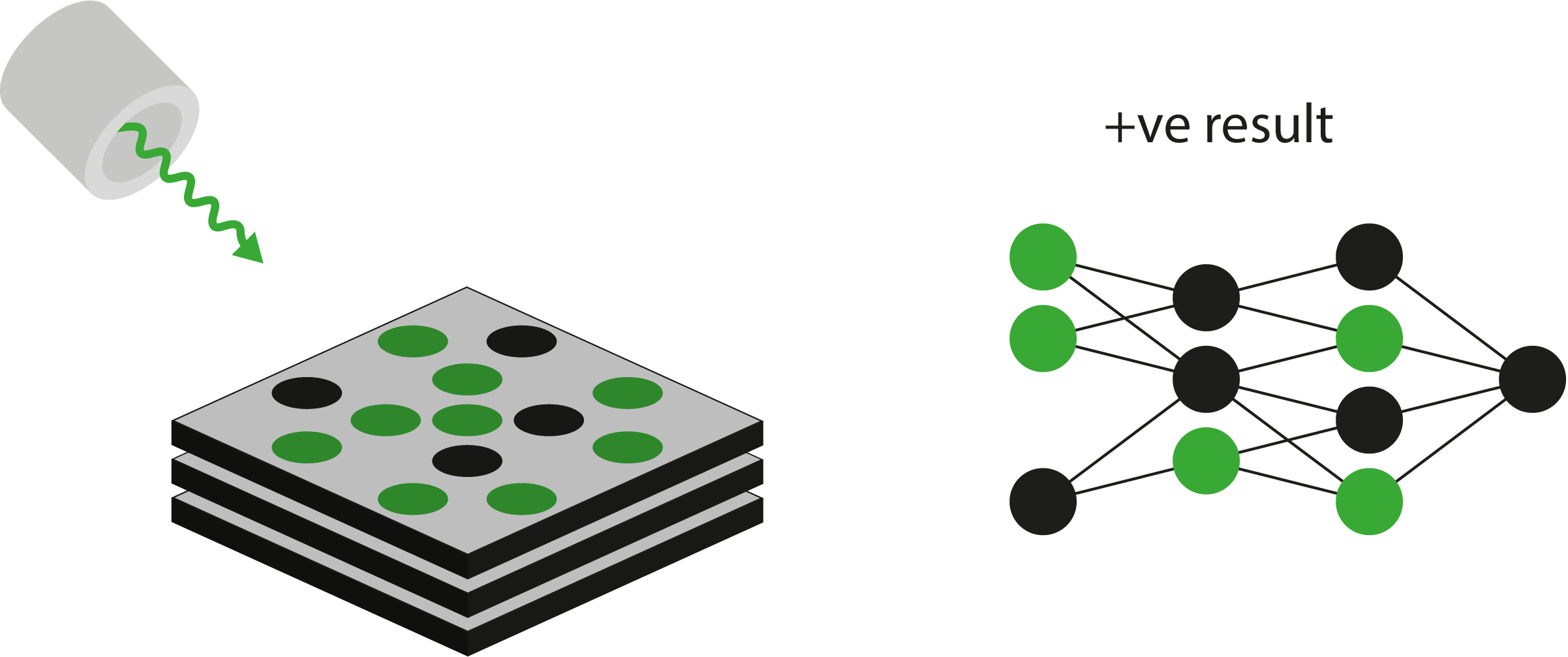
Figure 4. Left: Diagram showing illumination of multi-antigen panel by fluorescence;
Right: Readout and analysis by deep learning algorithm to diagnose infection. Adapted from Joung and Colleagues [18].
The advantage of such platforms is that high diagnostic specificity can be attained by requiring positive results for multiple antigen targets, with the ability to further optimize antigen selection and fine-tune diagnostic performance over time [20]. Furthermore, while many multiplex assays are not strictly usable at the point of care (PoC), the small plastic cassettes that many of them use in analysis, facilitates the decentralization of testing and transport of patient samples. However, several challenges still remain.
One of the biggest drawbacks of serological diagnosis is the difficulty in detecting IgM and IgG antibodies during the early stages of infection. While there are inherent delays as the body seroconverts, improving diagnostic sensitivity to detect lower antibody concentrations can significantly improve outcomes for patients in the early phase of infection. One way in which researchers have sought to do this, is by augmentation of antigen-antibody interactions. Lerner and colleagues, for example, attached antigens to the surface of carbon nanotubes to increase surface area for antibody adsorption, using transistors to detect voltage changes resulting from binding events [22]. The sensor effectively detected Borrelia antigens at concentrations as low as 1 ng/ml. Furthermore, the response varied over a range of concentration (up to 3µg/mL), conferring the assay with a degree of quantitative analysis. Other studies have successfully investigated the use of microbeads and microfluidic chips to improve binding interactions with similar success [23].
A promising means of overcoming the seroconversion gap altogether, has been with the detection of human biomarkers, such as mRNA, proteins or metabolites that correlate with Borrelia infection. Unlike antibodies for example, inflammatory markers appear almost instantaneously after Borrelia infection and may provide unique signatures of Borrelia infection [24]. Furthermore, by integrating these markers in quantitative, multiplexed assays, it may be possible to differentiate between active and past infection, as well as characterizing responses to antibiotic treatment [25].
Future Steps
In spite of its inherent limitations, 2TT is currently the only validated method for Lyme disease diagnosis [26]. Therefore, the development of multiplex platforms that can detect anti-Borrelia antibodies and biomarkers of active infection at the PoC would represent major advances in the field. However, further work is still needed to optimise these platforms for commercialisation – specifically in relation to their sensitivity, specificity and overall reliability. In particular, assay developers will need to provide more comprehensive performance data to establish that their assays are equivalent to, or better than, the current reference standard.
To support the ongoing research and development of next-generation diagnostics, The Native Antigen Company offers an extensive range of native and recombinant antigens and highly specific antibodies for multiple Borrelia species.
References
- Sanchez, E., Vannier, E. and Wormser, GP. et al. Diagnosis, Treatment, and Prevention of Lyme Disease, Human Granulocytic Anaplasmosis, and Babesiosis. JAMA. 2016;315(16):1767-1777. DOI: 1001/jama.2016.2884.
- Eldin, C., Raffetin, A. and Bouiller, K. et al. Review of European and American guidelines for the diagnosis of Lyme borreliosis. Medecine et Maladies Infectieuses. 2019;49(2):121-132. DOI: 1016/j.medmal.2018.11.011.
- Dumic, I. and Severnini, E. “Ticking Bomb”: The Impact of Climate Change on the Incidence of Lyme Disease. Canadian Journal of Infectious Diseases and Medical Microbiology. 2018:1-10. DOI: 1155/2018/5719081.
- Kurokawa, C., Lynn, GE. and Pedra, JHF. Interactions between Borrelia burgdorferiand ticks. Nat Rev Micro. 2020.
- How many people get Lyme disease? Centers for Disease Control and Prevention (CDC). https://www.cdc.gov/lyme/stats/humanCases.html. Accessed August 25th, 2020.
- European Centre for Disease Control and Prevention (ECDC). https://www.ecdc.europa.eu/en/borreliosis. Accessed August 25th, 2020.
- DeLong, A. Hsu, M. and Kotsoris, H. Estimation of cumulative number of post-treatment Lyme disease cases in the US, 2016 and 2020. BMC Public Health. 2019;19(352):1-8. DOI: 1186/s12889-019-6681-9.
- Keller, A., Graefen, A. and Ball, M. New insights into the Tyrolean Iceman’s origin and phenotype as inferred by whole-genome sequencing. Nat Comms. 2012;3(698):1-9. DOI: 1038/ncomms1701.
- Stone, BL., Tourand, Y. and Brissette, CA. Brave New Worlds: The Expanding Universe of Lyme Disease. Vector Borne Zoonotic Dis. 2017;17(9): 619–629. DOI: 1089/vbz.2017.2127.
- Aronowitz, RA. The Rise and Fall of the Lyme Disease Vaccines: A Cautionary Tale for Risk Interventions in American Medicine and Public Health. The Milbank Quarterly. 2012;90(2):250–277. DOI: 1111/j.1468-0009.2012.00663.x.
- Nigrovic, LE., Lewander, DP. and Balamuth, F. The Lyme Disease Polymerase Chain Reaction Test Has Low Sensitivity. Vector Borne Zoonotic Dis. 2020;20(4):310-313. DOI: 1089/vbz.2019.2547.
- Mead, P., Petersen, J. and Hinckley A. Updated CDC Recommendation for Serologic Diagnosis of Lyme Disease. CDC MMWR. 2019;68(32):703. https://www.cdc.gov/mmwr/volumes/68/wr/mm6832a4.htm
- Two-tiered Testing Decision Tree. Centers for Disease Control and Prevention. https://www.cdc.gov/lyme/healthcare/clinician_twotier.html. Accessed August 25th, 2020.
- Aguero-Rosenfeld, ME., Wang, G. and Schwartz, I. et al. Diagnosis of Lyme Borreliosis. Clin Microbiol Rev. 2005;18(3):484–509. DOI: 1128/CMR.18.3.484-509.2005.
- Merilainen, L, Brander, H. and Herranen, A. et al. Pleomorphic forms of Borrelia burgdorferiinduce distinct immune responses. Microbes and Infection. 2016;18(7-8):484-495. DOI: 1016/j.micinf.2016.04.002.
- Aguero-Rosenfeld, ME., Nowakowski, J. and Bittker, S. Evolution of the serologic response to Borrelia burgdorferi in treated patients with culture-confirmed erythema migrans. Journal of Clinical Microbiology. 1996;34(1):1-9. https://jcm.asm.org/content/34/1/1
- Marques, AR. Laboratory Diagnosis of Lyme Disease – Advances and Challenges. Infect Dis Clin North Am. 2015;29(2):295-307. DOI: 1016/j.idc.2015.02.005.
- Joung, H-A., Ballard, ZS. and Wu, J. et al. Point-of-Care Serodiagnostic Test for Early-Stage Lyme Disease Using a Multiplexed Paper-Based Immunoassay and Machine Learning. ACS Nano. 2020;14(1):229–240. DOI: 1021/acsnano.9b08151
- Cameron, DJ. Consequences of treatment delay in Lyme disease. J Eval Clin Pract. 2007;13(3):470-2. DOI: 1111/j.1365-2753.2006.00734.x.
- Lahey, LJ., Panas, MW. and Mao, R. et al. Development of a Multiantigen Panel for Improved Detection of Borrelia burgdorferi Infection in Early Lyme Disease. J Clin Microbiol. 2015;53(12):3834-3841. DOI: 1128/JCM.02111-15.
- Wormser, GP., Schriefer, M. and Aguero-Rosen, ME. et al. Single-Tier Testing with the C6 Peptide ELISA Kit Compared with Two-Tier Testing for Lyme Disease. Diagn Microbiol Infect Dis. 2013;75(1):9-15. DOI: 1016/j.diagmicrobio.2012.09.003.
- Lerner, MB., Dailey, J. and Goldsmith, BR. et al. Detecting Lyme disease using antibody-functionalized single-walled carbon nanotube transistors. Biosensors and Bioelectronics. 2013;45(163):163-167. DOI: 1016/j.bios.2013.01.035.
- Hilton, E., DeVoti, J. and Benach, JL. et al. Seroprevalence and seroconversion for tick-borne diseases in a high-risk population in the northeast United States. Am J Med. 1999;106(4):404-409. DOI: 1016/s0002-9343(99)00046-7.
- Soloski, MJ., Crowder, LA. and Lahey, LJ. et al. Serum inflammatory mediators as markers of human Lyme disease activity. PLoS One. 2014;9(4). DOI: 1371/journal.pone.0093243.
- Chou, E., Lasek-Nesselquist, E. and Taubner, B. A fluorescent plasmonic biochip assay for multiplex screening of diagnostic serum antibody targets in human Lyme disease. PLoS One. 2020;15(2). DOI: 1371/journal.pone.0228772.
- Schoen, RT. Challenges in the Diagnosis and Treatment of Lyme Disease. Curr Rheumatol Rep. 2020;22(1):3. DOI: 1007/s11926-019-0857-2.