Ticks are responsible for a diverse group of neglected, and rapidly expanding diseases, affecting humans, companion animals and livestock. A growing understanding of tick-host ecology, disease diversity, and their growing burden on human and animal health has demanded more integrative approaches to their diagnosis and control.
Tick Emergence and Distribution
Ticks are obligate ectoparasites of the Arachnida class, meaning they are more closely related to spiders and mites than insects. As haemoparasites, ticks feed on the blood of mammals, birds and reptiles to meet their nutritional requirements and progress through their lifecycles. After transferring to a host, a tick will locate a feeding spot, grasp the skin and lacerate the epidermis with their hypostome (harpoon-like appendage). The tick will then excrete anti-coagulants and anti-immune modulators to facilitate feeding and nullify the host’s immune response (Figure 1). Hard ticks of the Ixodidae family feed on their hosts for multiple days at a time before detaching, while soft ticks of the Argasidae family can complete feeding within a matter of minutes. Regardless, ticks can draw immense quantities of blood, equivalent to 200–600 times their unfed bodyweight.1
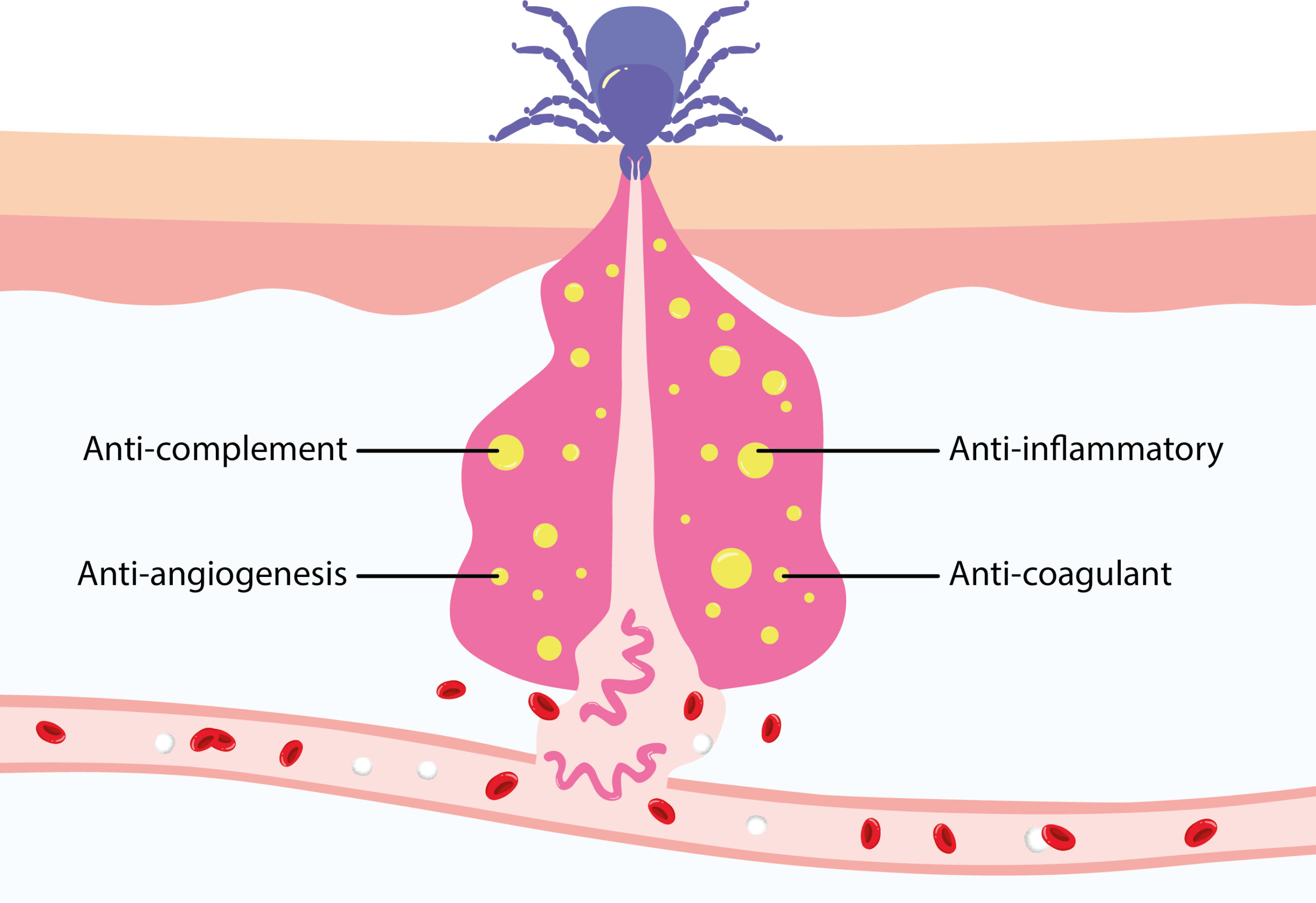
Figure 1: Diagram of tick feeding, releasing immune evasion factors and Borrelia spirochetes. Adapted from Kurokawa and colleagues.2
In addition to satisfying their nutritional requirements, this feeding process makes ticks ideal vectors of pathogens, which readily migrate from the tick’s gut to the host feeding site. Tick-borne pathogens (TBPs) have likely existed for millennia, evidenced by the genome of a 5,000-year-old Tyrolean ‘iceman’, containing Borrelia-like DNA (Figure 2).3 In spite of their long history, our discovery and understanding of TBPs is fairly recent, with the Rickettsia genus of bacteria being the first identified in the early 1900s. Over the last century, a constellation of bacterial, viral and protozoal pathogens have since been associated with ticks, responsible for a diverse range of diseases affecting human and animal hosts.
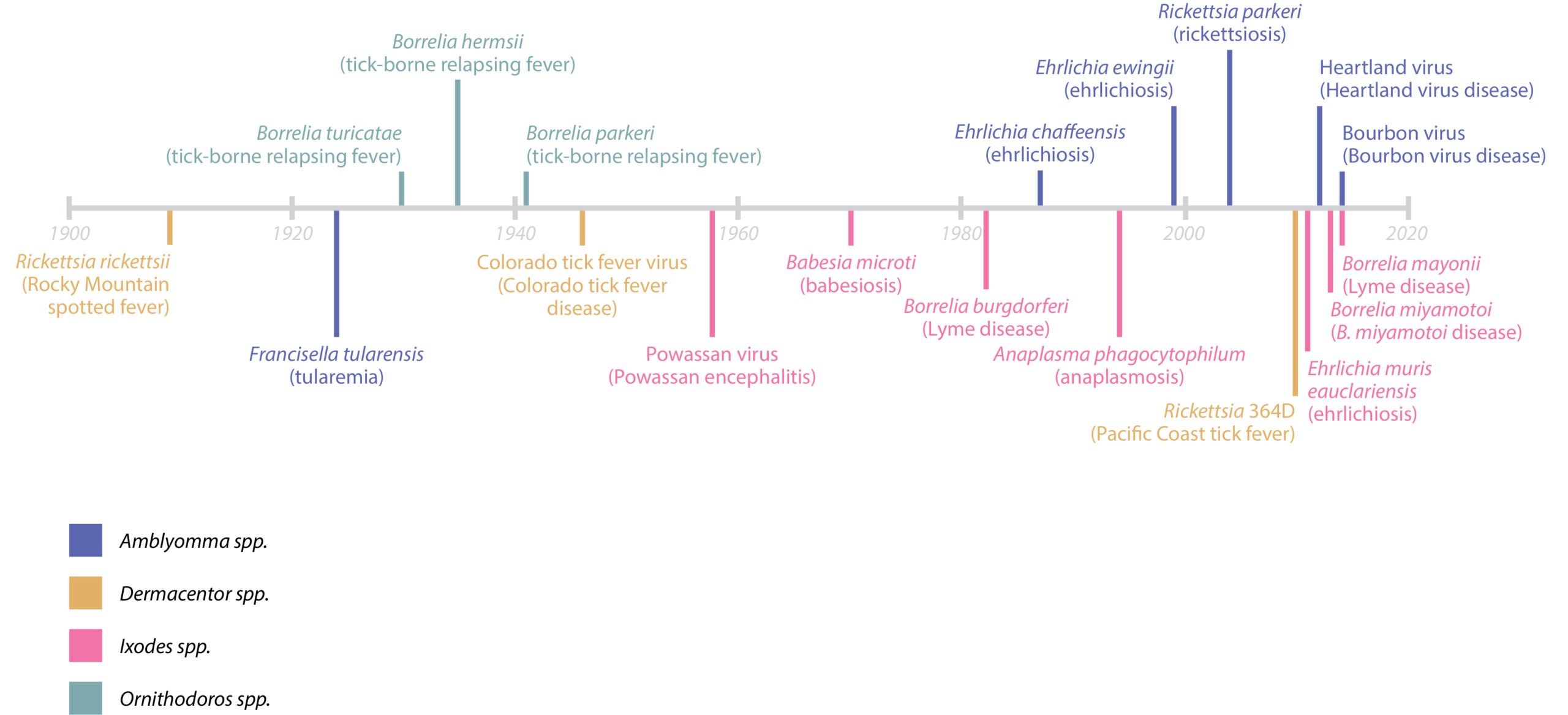
Figure 2. Timeline of discovery. Adapted from Eisen and Paddock4
The diseases that TBPs cause are highly diverse, manifesting in a range of acute and chronic pathologies, including fever, rash, headache, anaemia, jaundice, haemoglobinuria, and anorexia to name a few. Aside from transmitting pathogens, ticks are also responsible for severe toxic conditions, irritation, secondary infections and physical damage associated with their bites.
The emergence of many TBPs in recent decades has coincided with the rapid spread of tick populations through woodland areas. As of today, tick-borne diseases (TBDs) are the most rapidly emerging diseases globally, second only to mosquito-borne diseases in their health burden. Lyme disease – one of the most well-known and well-funded TBDs – accounts for approximately 600,000 new cases in the US and Europe each year, with 1–3 million people thought to be currently suffering from long-term effects in the US alone.5,6 For pets and livestock, TBDs are less-well characterised, though it is well-established that TBDs are major parasites of dogs and to a lesser extent, cats. For livestock, TBDs are the most significant vector-borne diseases affecting cattle and sheep, not only impacting wool, milk and meat productivity, but leading to significant population losses – a particular issue in resource-limited communities that are highly dependent on their productivity.7
One Health, Surveillance and Clinical Diagnosis
Historically, the paradigm for infectious diseases has viewed a subset of vectors that carry a single pathogen which causes a defined spectrum of disease. However, when considering the diversity of tick species, variability and overlap in their distribution, and diversity of co-circulating/infecting pathogens, this model falls short. Complicating matters, the constellation of animal, bird and reptile hosts that ticks and their pathogens infect play important ecological roles and serve as reservoirs that promote pathogen persistence and transmission. Combined with the pace of climate change, population growth, increased transportation, tourism and trade, traditional means of understanding TBP emergence and transmission are highly limited. As such, while considerable progress has been made in our understanding of TBDs in recent years, their complexity and interconnectedness have made disease control highly challenging. Indeed, TBDs are increasingly regarded as a neglected set of diseases, in both the appreciation of their clinical significance and expenditure in developing countermeasures.
To address this, more integrative approaches to TBD control are needed. In this respect, the One Health paradigm has gained much attention, for its view of integrated human, veterinary and environmental health (Figure 3). Though a broad concept, One Health can be viewed as a collaborative, transdisciplinary approach that aims to achieve optimal health outcomes at the local, national, and global levels by recognising the interconnection between people, animals, and their shared environment.8
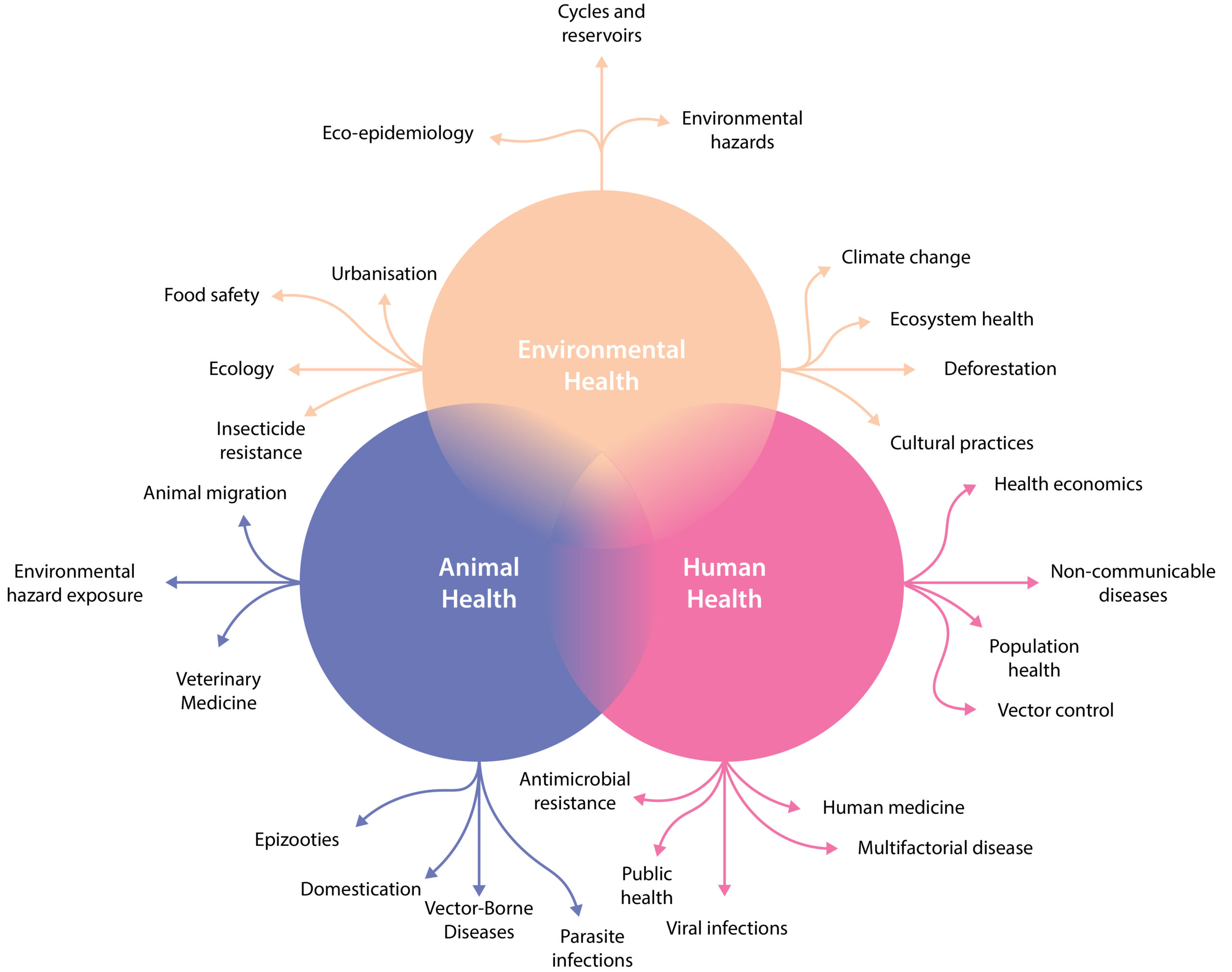
Figure 3. One Health scheme. Reproduced from Malliaraki, 20209
While a relatively new term, the concept of One Health has long been appreciated, tracing back as far as the ancient Greek physician, Hippocrates. For TBDs, there are many potential benefits to applying a One Health paradigm. A major hurdle is the natural division between human and veterinary medicine, which has resulted in a fragmented patchwork of disease surveillance, communication and control systems.1 Companion animals and livestock, for example, often act as sentinels for TBDs, meaning that veterinarians have the opportunity to play a decisive role in notifying public health authorities of outbreaks. Likewise, clinicians and veterinarians dealing with infection should not only treat the owner or animal, but input the data into a shared database to inform disease risk and treatment. This bilateral approach to the exchange of information would not only inform and expedite treatment decisions, but improve understanding of TBD pathologies and their distribution. The integration of such data with ecological surveillance could then inform further disciplines, including entomology, epidemiology and public health to improve control strategies and raise regional public awareness (Figure 4).10
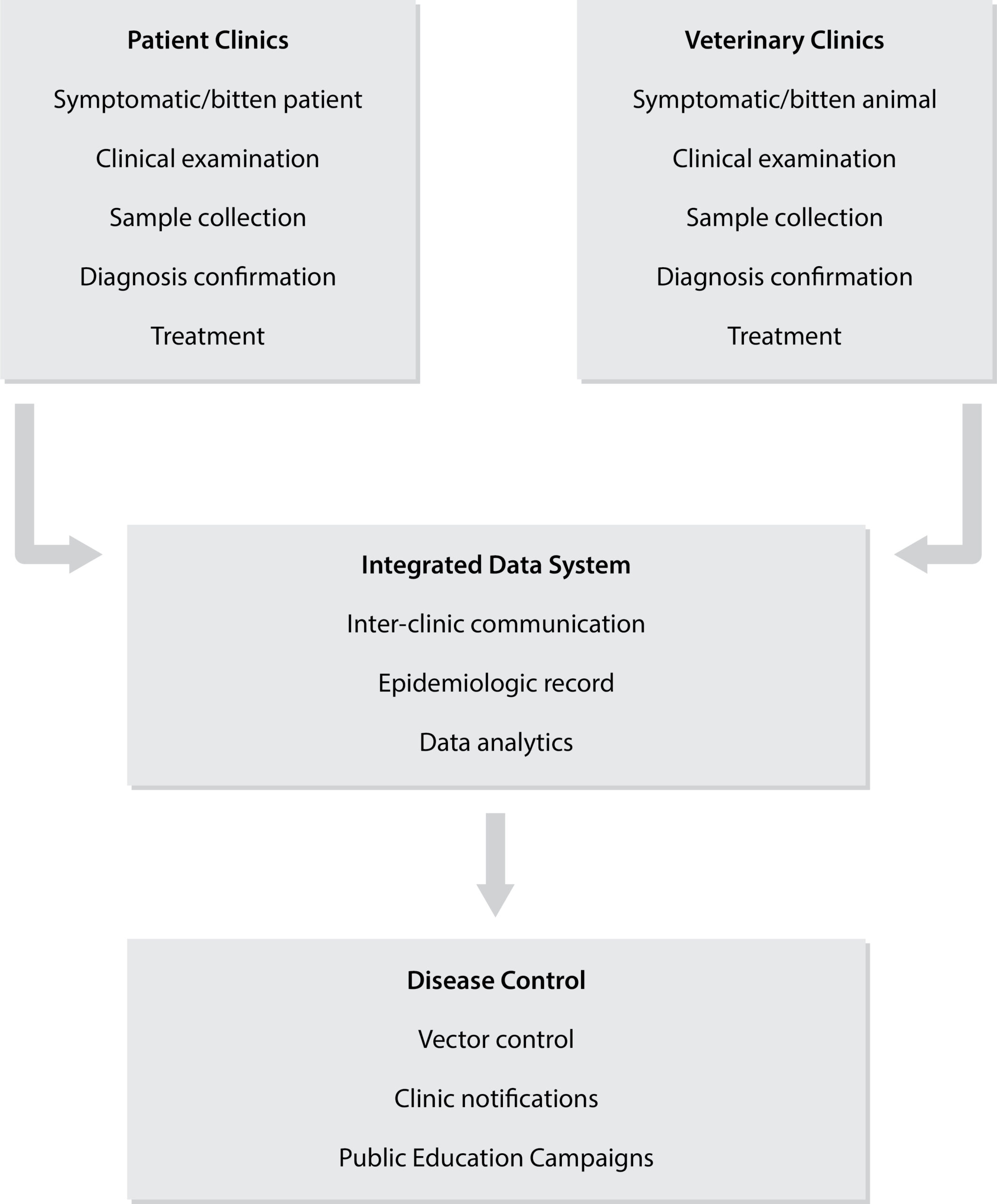
Figure 4. Diagram of potential human-veterinary sharing of TBD data.
The Need for Multiplexed, Point-of-Care Diagnostics
The quality, diversity and availability of diagnostic technologies have all improved tremendously in recent decades, bringing significant improvements to our understanding of, and the ability to treat infectious diseases. However, for TBDs, their relatively recent characterisation, resource limitations and ecological complexity has made diagnosis and surveillance an ongoing challenge.
Take Lyme disease (Borrelia) for example: it is one of the best characterised TBDs, with significant public health attention. However, due to its diverse clinical presentation, it can easily escape recognition in the absence of its distinctive erythema migrans rash. While multi-systemic in nature, Lyme bacteraemia is also highly transient after initial infection, making isolation for culture, microscopic examination or PCR unreliable.11 As such, serology has so far proven to be the only reliable option, yet requires two separate ELISA tests which often achieve less than 40% sensitivity in the first 3 weeks of infection when antibiotic treatment is most effective.12
For patients, these inaccurate or delayed diagnoses risk the development of more severe and chronic forms of disease. Indeed, many unexplained syndromes associated with tick bites have led to considerable discord between infectious disease institutions and patient associations.13 For public health authorities, these limitations result in patchy datasets, subject to significant spatial and temporal bias that make transmission dynamics, outbreak risk and geographic disease burden challenging to estimate.
Therefore, to establish the groundwork for a One Health approach to TBDs, diagnostic improvement is a major goal. This challenge is in no small part, reflected by the diverse range of bacterial, viral and protozoal TBPs, responsible for a multitude of acute and chronic pathologies, which in turn have necessitated a battery of visual, molecular, serological, cell culture and immuno-histochemical tests. Yet, given the limitations of existing diagnostics and growing threat that TBDs pose, there is an increasing consensus that new generation technologies are needed. This challenge can be broken down into two main areas: performance and accessibility.
The performance of any diagnostic is highly dependent on the nature of disease and methodology used. However, given the prevalence of co-circulating TBPs, reliance on reported tick bite exposures and identification of generic and overlapping symptoms, there is a clear need for multiplexed assays that can test for a broader range of pathogens. Here, many molecular diagnostic methods, including next-generation sequencing, metagenomics and PCR promise improved detection of novel and emerging pathogens with the ability to detect a litany of targets within a single assay. A promising platform are micro-arrays, which can simultaneously capture and detect thousands of molecular and protein analytes.14 In combination with digital readers that employ machine learning, these tests have shown to achieve significantly better sensitivities and specificities, with added advantage of accommodating analyte selection to fine-tune performance over time.
For chronic infections such as Lyme disease and tick-borne encephalitis, markers of disease may wax and wane as pathogens resurge and re-stimulate the immune system (Figure 5). However, as these windows of diagnostic opportunity are unpredictable and short-lived, multiple diagnostic technologies need to be integrated to maximise the sensitivity of detection. A promising means of overcoming this challenge altogether has been with the detection of host biomarkers, such as mRNAs, proteins or metabolites that act as general correlates of infection.
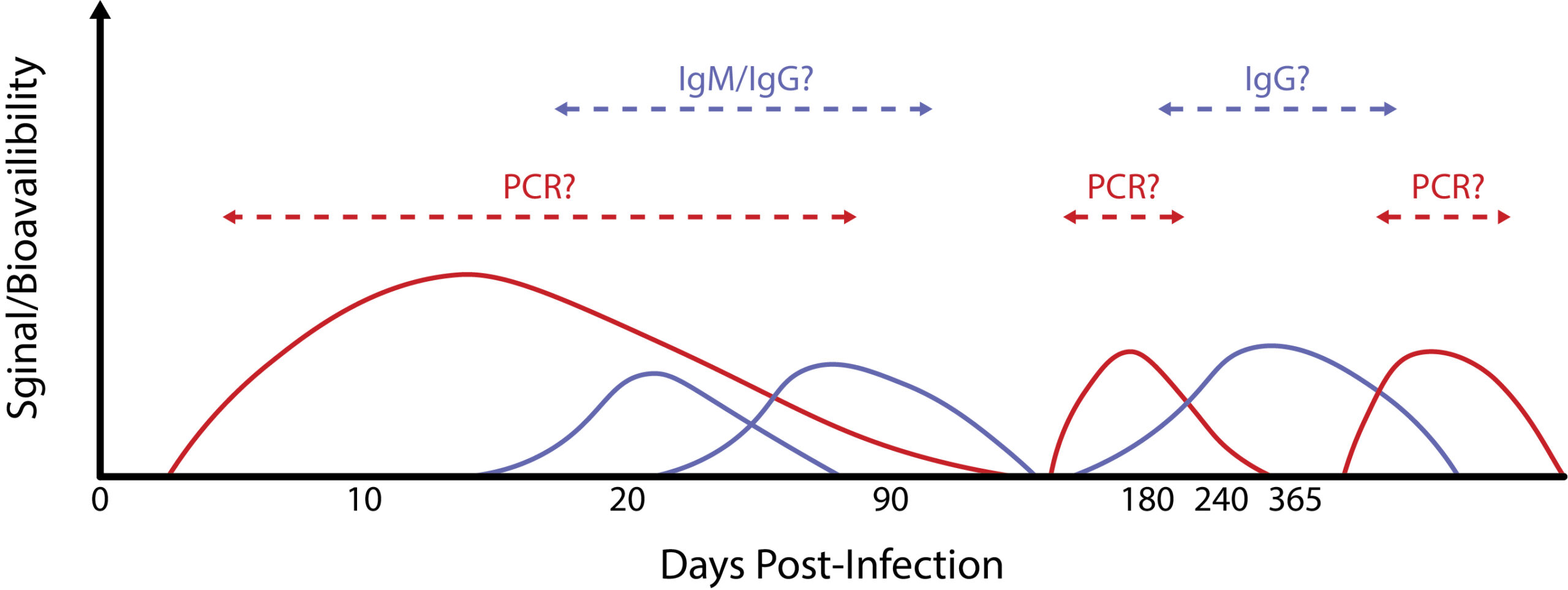
Figure 5. Hypothetical scheme of PCR/antibody detection windows for chronic tick-borne disease.
The availability of increasingly sophisticated diagnostic tools has revolutionised our capacity to detect and respond to health threats. Yet, no matter how sophisticated, the initial identification of pathogens begins at the local community level where human-animal cases occur. Unfortunately, many areas at high TBD risk lack the necessary infrastructure and expertise to support robust laboratory diagnostic systems, meaning TBD testing is largely restricted to clinical-veterinary laboratories in urban areas, far away from the primary interfaces of human-animal-tick interactions.
To address this, the development of point-of-care (PoC) platforms are needed that can be distributed to at-risk settings to improve the availability and speed of diagnosis in order to support and span the full diagnostic spectrum. However, as the majority of existing PoC platforms are ‘singleplex’ by nature, this in turn creates further challenges. One way of meeting this balance is the use of plastic cassettes that can be easily transported to laboratory facilities to decentralise testing. Alternatively, multiplexed PoC platforms, such as vertical flow assays can be used to detect a range of analytes.15
References
1. Dantas-Torres, F. and Otranto, D. Best Practices for Prevent-ing Vector-Borne Diseases in Dogs and Humans. Trends in Parasitol. 32(1), 43–55 (2016).
2. Kurokawa, C. et al. E. Interactions between Borrelia burgdorferi and ticks. Nat. Rev. Micro. 18, 587–600 (2020).
3. Keller, A. et al. New insights into the Tyrolean Iceman’s origin and phenotype as inferred by whole-genome sequencing. Nat. Comms. 3(698) (2021).
4. Eisen, R.J. & Paddock, C.D. Tick and Tickborne Pathogen Surveil-lance as a Public Health Tool in the United States. J. Med. Entomol. 1–13 (2020).
5. https://www.euro.who.int/__data/assets/pdf_file/0008/246167/Fact-sheet-Lyme-borreliosis-Eng.pdf, visited 24 Jun 2021.
6. DeLong, A., Hsu, M. & Kotsoris, H. Estimation of cumulative number of post-treatment Lyme disease cases in the US, 2016 and 2020. BMC Pub. Health. 19(352) (2019).
7. Salih, D.A., Hussein, A.M. & Singla, LD. Diagnostic approaches for tick-borne haemoparasitic diseases in livestock. Vet. Med. and Ani. Hlth. 7(2), 45–56 (2015).
8. https://www.cdc.gov/onehealth/index.html, visited 24 Jun 2021.
9. https://eirinimalliaraki.medium.com/one-health-may-pre-vent-the-next-pandemic-a83c3465ccab, visited 24 Jun 2021.
10. Baneth, G. Tick-borne infections of animals and humans: a common ground. Int. J. Parasitol. 44(9), 591–6 (2014).
11. Nigrovic, L.E. et al. The Lyme Disease Polymerase Chain Reaction Test Has Low Sensitivity. Vector Borne Zoonotic Dis. 20(4), 310–313 (2020).
12. Aguerio-Rosenfeld, M.E. et al. Diagnosis of Lyme Borreliosis. Clin. Microbiol. Rev. 18(3), 484–509 (2005).
13. Vayssier-Taussat, M., et al. How a multidisciplinary ‘One Health’ approach can combat the tick-borne pathogen threat in Europe. Future Microbiol. 10(5), 809–818 (2015).
14. Jayaraman, V. et al. An ultra-high-density protein microarray for high throughput single-tier serological detection of Lyme disease. Sci. Rep. 10(18085) (2020).
15. Joung, H-A. et al. Point-of-Care Serodiagnostic Test for Early-Stage Lyme Disease Using a Multiplexed Paper-Based Immunoassay and Machine Learning. ACS Nano. 14(1), 229–240 (2020).