This article has been published in Volume 2, Issue 3 of the IBI journal.
While Zika is no longer in the public eye, it hasn’t vanished. Recent outbreaks across Asia and Africa are reminders that Zika is alive and well, and with no effective countermeasures available, little can be done for mothers at risk of infection. A major gap in our protective arsenal is a lack of sensitive and accurate diagnostics. Immunoassay development has been severely hampered by cross-reactive antibodies, requiring new approaches to the production of reagents and assay design.
The 2015 Epidemic
In the spring of 2015, north-eastern Brazil was hit by a mysterious disease. Doctors’ reports described an illness characterised by malaise, rash, fever and a headache, but with mild symptoms and no deaths directly attributable. It was a powerful El Niño year and the humid weather had caused a boom in mosquito populations that had tripled the expected cases of dengue fever and in August, reports of microcephaly – a condition in which the heads of newborns are abnormally small – were mounting in the city of Recife. Zika soon exploded in Brazil, spreading through much of South and Central America in the months that followed [1]. Health organisations hurried to contain the outbreak and in February 2016, the World Health Organization declared the situation to be a Public Health Emergency of International Concern (PHEIC) [2]. By the height of the epidemic, 200,000 people had been infected and thousands of babies were born with severe birth defects [3]. The economic toll was equally as devastating, with estimates as high as US$18 billion lost from tourism and healthcare services [4]. Through a combination of international support and growing levels of immunity, the epidemic was brought under control by the end of the summer and the global incidence of Zika infection has since decreased.
Zika Virus Infection
Zika virus (ZIKV) is single-stranded RNA virus of the Flaviviridae family, which comprises some of the world’s major mosquitoborne pathogens, including dengue, yellow fever and the West Nile virus. ZIKV is spread by the Aedes genus of mosquitos, which carries the virus and transmits it to the host’s bloodstream when feeding. Most of those infected with ZIKV show no symptoms, however, pregnant women are able to transmit the virus to the developing foetus, which can impede neurological development, resulting in a spectrum of abnormalities known as congenital Zika syndrome (CZS) [5]. The most well-known outcomes of CZS include microcephaly, mental disabilities and blindness. In rare cases, ZIKV can also cause an autoimmune disorder known as Guillain-Barré syndrome (GBS), whereby the immune system goes into overdrive, attacking the body’s peripheral nerves. This results in muscle weakness, flaccid paralysis and in some cases, death [6].
Why Zika is Still a Threat
The 2015 Zika epidemic was a boon for academia and industry, alike. Scientists quickly refocused their research efforts in large multidisciplinary collaborations and made tremendous progress in a short space of time [7]. Numerous vaccine and therapeutic candidates were evaluated in non-clinical studies and many have since moved into development [8]. However, there are still significant gaps in our understanding of ZIKV, and no effective countermeasures to Zika infection. This leaves millions of women at risk of infection, with no options or treatment other than relieving symptoms. Moreover, in the coming years, the habitats of the Aedes mosquitos are expected to grow as global temperature rise, further expanding into North America, southern Europe, southern Africa and the Middle East. Models estimate that as much as 50% of the world’s population could be at risk of ZIKV infection by 2050 as a result [9].
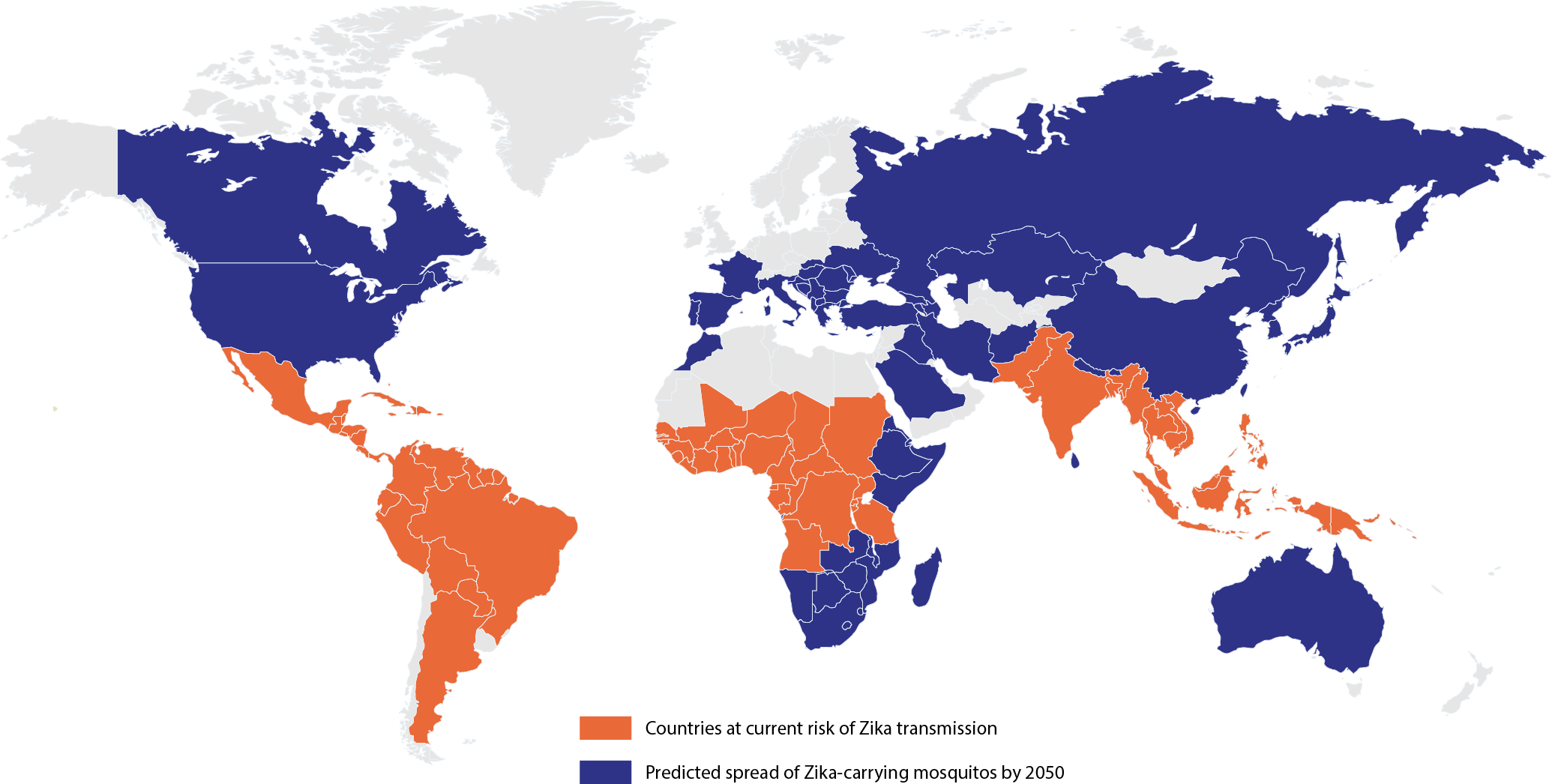
Map of current and predicted distribution of Zika virus by 2050. [9,10]
Controlling Zika
Given the serious human health risks that ZIKV poses and its profound socioeconomic impact, there is a need for academics and researchers to work towards more effective control strategies. In addition to developing vector control technologies, vaccines, and therapeutics, there is a pressing need for better means of diagnosis.
Many of the countries where Zika is known to circulate lack the necessary surveillance and healthcare infrastructure for case identification, which leaves patchy and incomplete data on ZIKV’s global distribution [11]. Moreover, as most of those infected don’t develop any symptoms, the majority of cases go unreported. Leading up to the 2015 epidemic, the virus had been circulating in the Americas for nearly two years before it was detected [12]. When symptoms do present, they tend to overlap with other mosquito-borne diseases, like malaria, dengue and chikungunya, which makes clinical evaluation highly inaccurate [12]. Combined, insufficient laboratory infrastructure, lack of symptoms, and overlapping sequelae makes routine testing with accurate diagnostics a necessity for case identification and epidemiological surveillance of ZIKV. In the long term, the best prospect for sustainably controlling ZIKV is with a safe and effective vaccine. Widespread administration of a vaccine has the potential to ensure that immunised women are no longer at risk of CZS however, the development of both diagnostics and vaccines for ZIKV have been fraught with difficulty.
The Cross-Reactivity Problem
Several viruses of the Flaviviridae family are closely related to one another and share a high degree of structural similarity. As a result, antibodies that bind to one of these viruses in the bloodstream can also recognise and bind other flaviviruses – a phenomenon known as cross-reactivity. In particular, the envelope proteins of ZIKV and the Dengue virus (DENV) are very structurally similar, so that serological (antibody-based) diagnostics are often unable to distinguish between the two viruses, giving positive results regardless of which virus has been detected [13]. This lack of diagnostic specificity poses a serious health risk to pregnant women, as mis-diagnosing ZIKV infection can result in incorrect evaluations of congenital disease risks, abortions, and unwarranted delays in pregnancy [13].
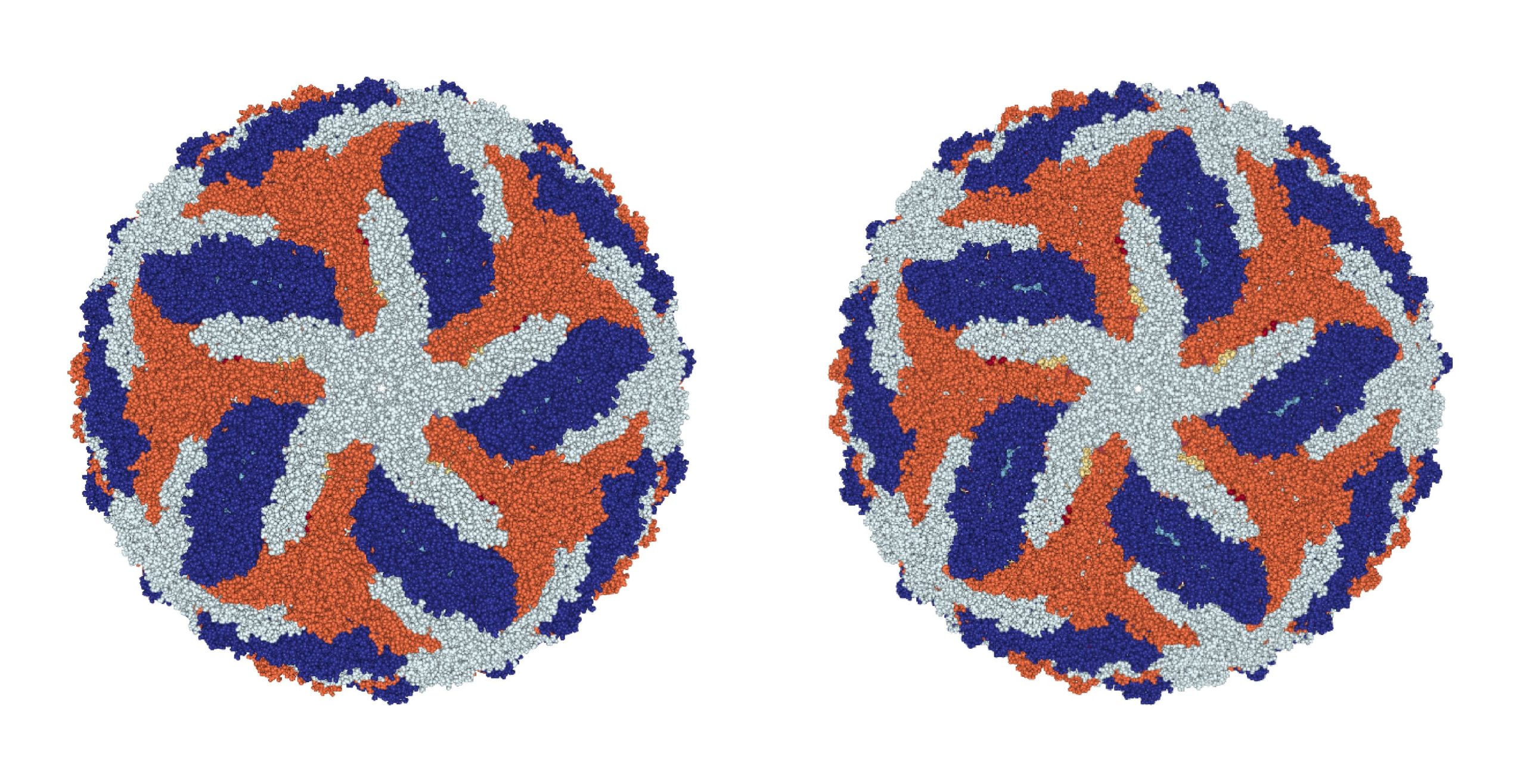
Spot the difference: Cryo-EM structures of the Zika and Dengue viruses. Left: Dengue cryo-EM structure [14]. Right: Zika cryo-EM structure [15].
For vaccines, cross-reactivity poses an equally substantial health risk. Cross-reactivity can cause a subsequent phenomenon known as antibody-dependent enhancement (ADE), whereby cross-reacting antibodies to one type of flavivirus are able to bind other types of flavivirus and enhance infection in the body. This is observed in natural infections when a patient who has been exposed to one DENV serotype is subsequently infected with another serotype and is at much greater risk of severe disease. Indeed, the first vaccine licensed for dengue, a live attenuated vaccine called Dengvaxia® (CYD-TDV) has suffered from this problem. Dengvaxia has proved to be safe and effective in seropositive people, but vaccination of seronegative individuals increases the risk of severe dengue following subsequent infection. Though Dengvaxia has been approved in 20 countries, its utility has been limited due to these safety issues. Therefore, in order to mitigate patient risk, pre-vaccine serological screening is crucial to ensure that only seropositive patients are vaccinated. For a Zika vaccine, the stakes are even higher, as the participation of pregnant women will be necessary to produce robust data on vaccine tolerance and safety [16]. As such, accurate serological diagnostics will be essential to distinguish previous ZIKV and DENV infections for safe vaccine trials.
Building Better Diagnostics
Developing accurate diagnostics for ZIKV has been challenged by the prevalence of cross-reacting antibodies in Zika and dengue-endemic populations. Likewise, developing effective vaccines against Zika has been impeded by the potential impact of enhanced pathogenicity in previously DENV-infected individuals. If a successful vaccine is developed, diagnosis of individuals will also be necessary to distinguish vaccine responses from actual infections with ZIKV, or other related viruses.
To address diagnostic cross-reactivity, various methods have been investigated. Classical enzyme-linked immunosorbent assays (ELISA) measure the binding of patient sera antibodies to antigens, immobilised in microtitre wells. However, traditional ZIKV antigens contain many cross-reacting epitopes with other flaviviruses, and assays with these antigens are frequently unable to reliably distinguish between flavivirus infections. This has prompted screening of ZIKV antigen arrays alongside mutation studies aimed at removing cross-reactive epitopes, while retaining ZIKV-specific regions. While no consensus has been reached regarding the ‘best’ antigens, recent findings suggest that the flaviviral NS1 protein is preferred for detecting specific IgG whilst the envelope protein may be needed to provide maximum sensitivity for detecting IgM responses.
The performance of immunoassays can also be improved. One approach is to coat anti-IgM onto a microtitre plate to capture IgM from patient sera and measure its reactivity with antigen (so-called μ-capture). However, such assays tend to be both complicated and lengthy. More recent formats include the ‘blockade of binding’ (BoB) assays, in which antibodies compete to bind antigen, double antigen bridging assays (DABA) that use a double antigen sandwich to increase specificity, and the use of ‘quenching’ antigens to ‘absorb-out’ cross-reactive antibodies.
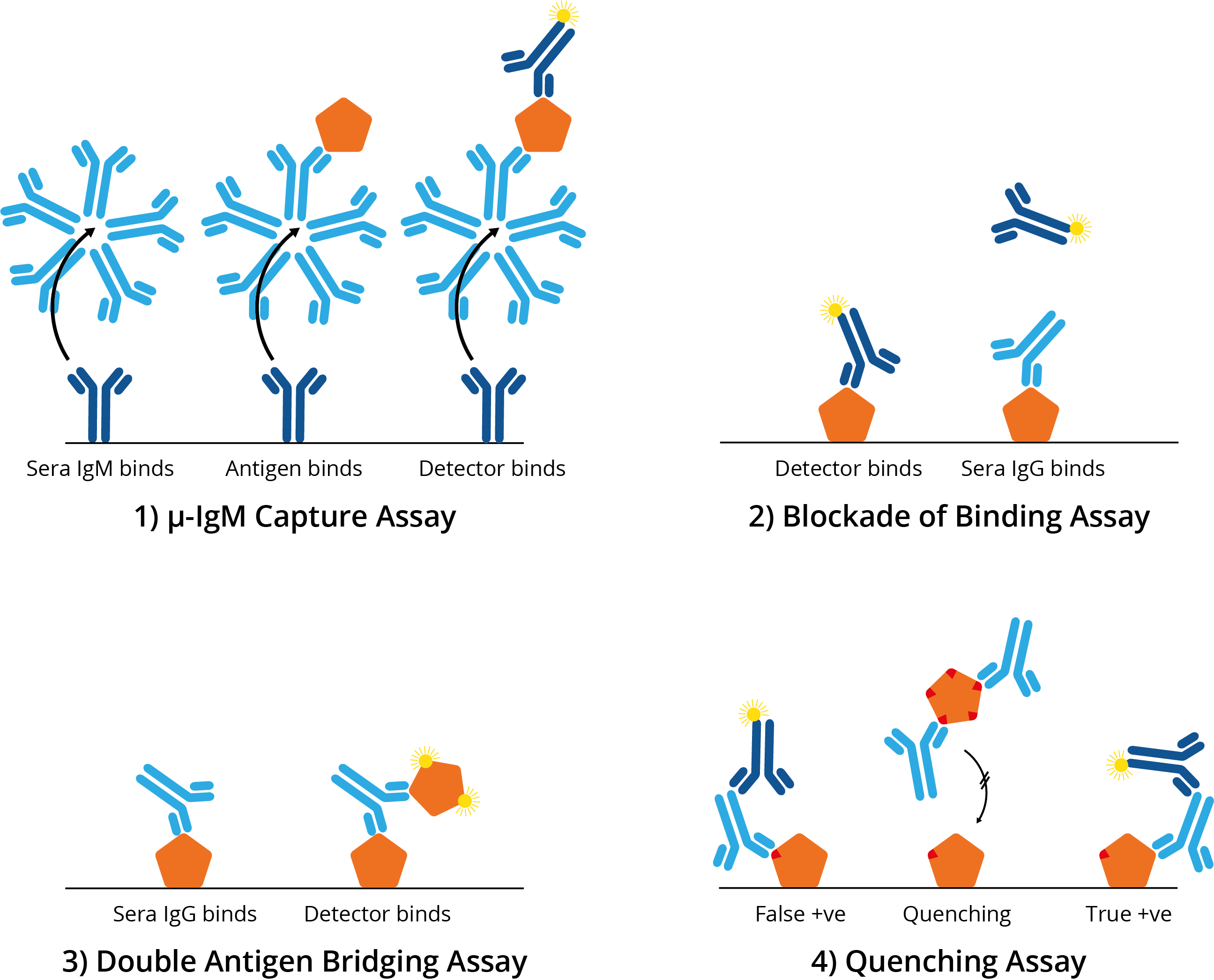
Four alternative ELISA formats to mitigate ZIKV cross-reactivity: 1) μ-IgM capture assay: IgM antibodies in patient sera bind to well-bound anti-human IgM. Once bound, antigen and then a detection antibody bind; 2) Blockade of binding assay: In the absence of patient antibody sera, a specific monoclonal antibody binds well-bound antigen and produces a signal. In the presence of patient antibody sera, patient antibodies bind well-bound antigen and exclude the detection antibody, resulting in a negative signal; The monoclonal antibody utilised binds a highly specific Zika epitope, and thus is only blocked by Zika-specific patient sera and not by cross-reactive dengue sera; 3) Double antigen bridging assay: Patient sera antibody binds well-bound antigen, followed by labelled detection antigen; This format inherently detects higher affinity antibodies with higher specificity; 4) Quenching assay: DENV patient sera antibodies are able to bind cross-reactive ZIKV antigen epitopes leading to false positive results. To prevent this, excess DENV antigen is added in solution to quench cross-reacting antibodies. This allows only ZIKV-specific antibodies to produce a true-positive signal.
Though these design strategies are far from exhaustive, they have shown some initial promise in mitigating cross-reactivity. Nonetheless, few have been extensively evaluated, and while there have been general improvements in assays that are commercially available, specificity claims are not always borne out of independent studies. As such, more rigorous diagnostic studies are needed to evaluate these designs and find practical solutions.
In Summary
Zika has proved to be a significant and ongoing threat to human health, with long-term consequences for the populations affected. In addition to a paucity of epidemiological data, there is a lack of existing countermeasures and treatments, which leaves millions at risk from future outbreaks. Advances in tackling ZIKV have been severely hampered by the cross-reactivity of anti-ZIKV/DENV antibodies, which have plagued both diagnostic and vaccine R&D. To overcome this, well-characterised antigen-antibody pairs, novel assay design strategies, and rigorous pre- and post-trial testing, in multiple populations will be required.
References
1. Salles, T. S., Sá-Guimarães, T. D. E., Alvarenga, E. S. L. D., Ribeiro, V. G., Meneses, M. D. F. D., Castro-Salles, P. F. D., Santos, C. R. D., Melo, A. C. D. A., Soares, M. R., Ferreira, D. F. & Moneira, M. F. History, epidemiology and diagnostics of dengue in the American and Brazilian contexts: a review. Parasit & Vectors. 11, 264 (2018).
2. https://www.who.int/en/news-room/detail/18-11-2016-fifthmeeting-of-the-emergency-committee-under-the-internationalhealth- regulations-(2005)-regarding-microcephaly-other-neurological-disorders-and-zika-virus, visited on 16 October 2019.
3. Lowe, R., Barcellos, C., Brasil, P., Cruz, O. G., Honório, N. A., Kuper, H. & Carvalho, M. S. The Zika Virus Epidemic in Brazil: From Discovery to Future Implications. Int. J. Environ. Res. Public Health. 15, 96 (2018).
4. Saeed, O., Afzal, M. R., Ahrar, A., Chughtai, M., Hassan, A., Ishfaq, M. F., Lobanova, I., Malik, M. I., Malik, A. A., Qureshi, M. A., Qureshi, I. A., Qureshi, M. H. & Saleem, M. A. Zika Virus Disease: From Origin to Outbreak. Academic Press. 137–142 (2018).
5. Ventura, C. V. & Ventura, L. O. Ophthalmologic Manifestations Associated With Zika Virus Infection. Pediatrics. 141 (2018).
6. Barbi, L., Coelho, A. V. C., Alencar, L. C. A. D. & Crovella, S. Prevalence of Guillain-Barré syndrome among Zika virus infected cases: a bsystematic review and meta-analysis. Braz. J. Infect. Dis. 22, 2 (2018).
7. Erbelding, E. & Cassetti, C. Zika Virus and Future Research Directions. J. Inf. Dis. 216, 10 (2017).
8. Wilder-Smith, A., Vannice, K., Durbin, A., Hombach, J., Thomas, S. J., Thevarjan, I. & Simmons, C. P. Zika vaccines and therapeutics: landscape analysis and challenges ahead. BMC Med. 16, 84 (2018).
9. Kraemer, M. U. G., Reiner, R. C., Brady, O. J., Messina, J. P., Gilbert, M., Pigott, D. M., Yi, D., Johnson, K., Earl, L., Marczak, L. B., Shirude, S., Weaver, N. D., Bisanzio, D., Perkins, T. A., Lai, Shengjie, Lu, S., Jones, P., Coelho, G. E., Carvalho, R. G., Bortel, W. V., Marsboom, C., Hendrickx, G., Schaffner, F., Moore, D. G., Nax, H. H., Bengtsson, L., Wetter, E., Tatem, A. J., Brownstein, J. S., Smith, D. L., Lambrechts, L., Cauchemez, S., Linard, C., Faria, N. R., Oliver, G. P., Scott, T. W., Liu, Q., Yu, H., Wint, G. R. W., Hay, bS. I. & Golding, N. Past and future spread of the arbovirus vectors Aedes aegypti and Aedes albopictus. Nat. Microbiol. 4, 854–863 (2019).
10. https://www.fertilitycenter.com/fertility_cares_blog/world-map-ofareas-with-risk-of-zika/, visited on 16 October 2019.
11. Plourde, A. R. & Bloch, E. M. A literature Review of Zika Virus. Emerg. Infect. Dis. 2016 July 22, 7.
12. Faria, N. R., Quick, J., Claro, I. M., Thézé, J., Jesus J. G. D., Giovanetti, M., Kraemer, M. U. G., Hill, S. C., Black, A., Costa, A. C. D., Franco, L. C., Silva, S. P., Wi, C-H, Raghwani, J., Cauchemez, S., Plessis, L. D., Verotti, M. P., Oliveira, W. K. D., Carmo, E. H., Coelho, G. E., Santellia, A. C. F. S., Vinhal, L. C., Henriques, C. M., Simpson, J. T., Loose, M., Andersen, K. G., Grubaugh, N. D., Somasekar, S., Chiu, C. Y., Muñoz-Medina, J. E., Gonzalez-Bonilla, C. R., Arias, C. F., Lewis-Ximenez, L. L., Baylis, S. A., Chieppe, A. O., Aguiar, S. F., Fernandes, C. A., Lemos, P. S., Nascimento, L. S., Monteiro, H. A. O., Siqueira, I. C., Queiroz, M. G. D, Souza, T. R. D, Bezerra, J. F., Lemos, M. R., Pereira, G. F., Loudal, D., Moura, L. C., Dhalia, R., França, R. F., Magalhães, T., Marques, E. T., Jaenisch, T., Wallau, G. L., Lima, M. C. D., Cerqueira, E. M. D., Lima, M. M. D., Mascarenhas, D. L., Neto, J. P. M., Levin, A. S., Tozetto-Mendoza, T. R., Fonesca, S. N., Mendes-Correa, M. C., Milagres, F. P., Segurado, A., Holmes, E. C., Rambaut, A., Bedford, T., Nunes, M. R. T., Sabino, E. C., Alcantara, L. C. J., Loman, N. J. & Pybus, O. G. Establishment and cryptic transmission of Zika virus in Brazil and the Americas. Nature. 15, 546 (2017).
13. Fischer, C., Drosten, C. & Drexler, F. J. The difficulties in obtaining reliable Zika virus diagnostics. Lancet Infect. Dis. 19, 240–241 (2019).
14. Zhang, X., Ge, P., Brannan, J. M., Zhang, Q., Schein, S. & Zhou, Z. H. Cryo-EM structure of the mature dengue virus at 3.5-Å resolution. Nat. Struct. Mol. Biol. 20, 105 (2013).
15. Sirohi, D., Chen, Z., Sun, L., Klose, T., Pierson, T. C., Rossmann, M. G. & Kuhn, R. J. The 3.8 Å resolution cryo-EM structure of Zika virus. Science. 22, 352 (2016).
16. Schwartz, D. A. Clinical Trials and Administration of Zika Virus Vaccine in Pregnant Women: Lessons (that Should Have Been) Learned from Excluding Immunization with the Ebola Vaccine during Pregnancy and Lactation. Vaccines. 6, 81 (2018).