In the first of a three-part series on the design, immunology and manufacture of COVID-19 vaccines, we take an early look at the major technologies under development and weigh-up the challenges these vaccines will face in reaching late-phase clinical trials.
Why We Need a Vaccine
Since its emergence in late 2019, SARS-CoV-2 has spread to over 200 countries, infecting over 2 million individuals and resulting in 150,000 deaths [1]. The novel coronavirus has proved to be a capable pathogen, balancing high transmissibility, with long incubation periods and a high prevalence of asymptomatic infection that have made it very difficult to contain.
For the most part, governments are doing what they can to implement non-pharmaceutical interventions, such as social distancing, self-isolation and the distribution of personal protective equipment (PPE) [2]. However, even at their most effective, containment efforts have only slowed the virus, and cannot prevent the inevitable rebounds as restrictions are lifted. And while there’s been plenty of hype about the use of off-label drugs, they have yet to show efficacy in controlled trials and risk of inducing harmful side effects [3].
The resulting socioeconomic consequences of the pandemic have been substantial. As governments are being forced to hamstring their economies and place over a third of the world’s population under lock-down, the global economy has entered a state of paralysis that risks one of the biggest recessions in a generation. Unemployment levels have spiked to near-record highs, we are unable to see friends or family, and many children are no longer receiving a full education.
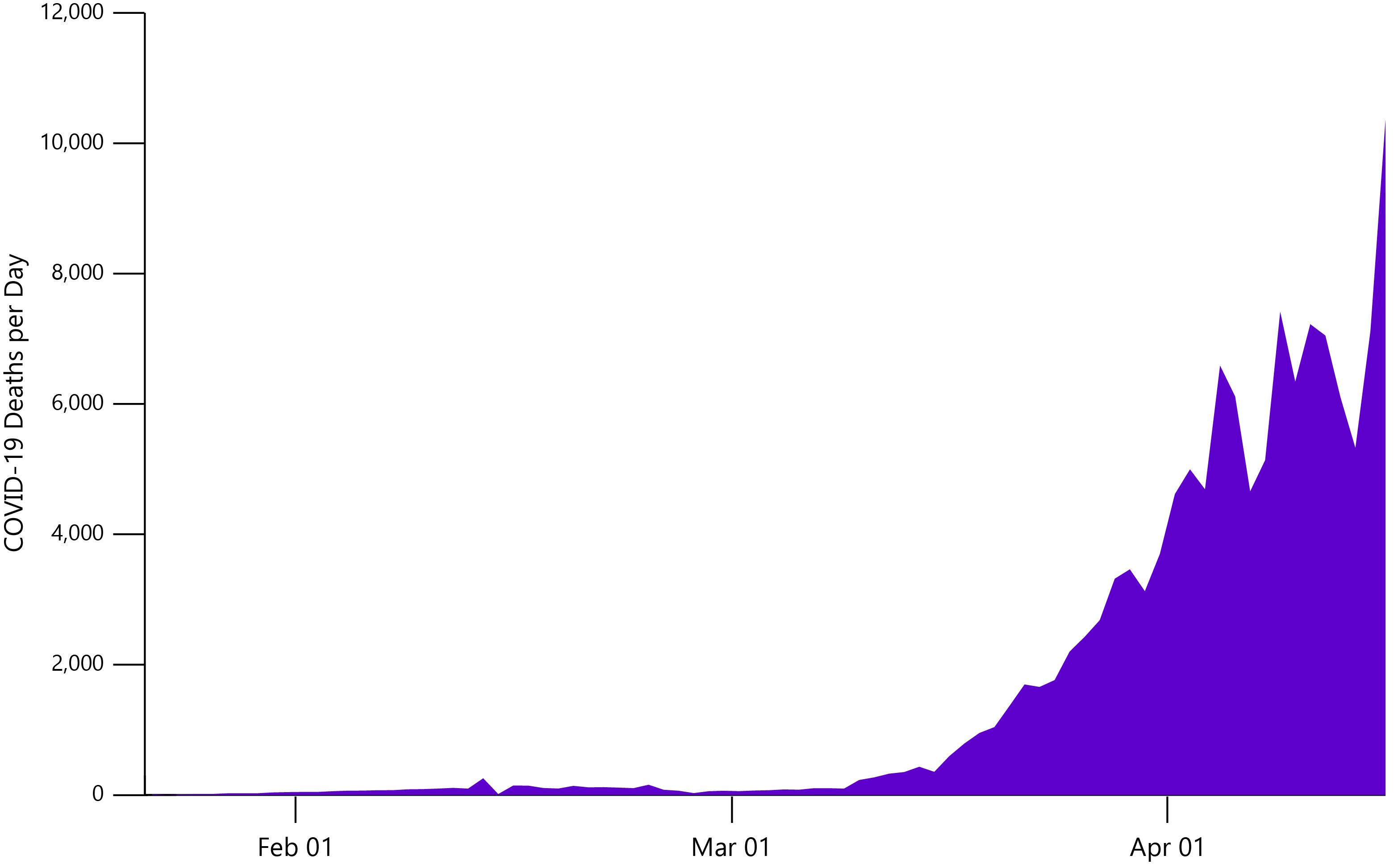
Confirmed COVID-19 deaths, worldwide from Jan 22 – Apr 16. Data from Our World in Data.
It goes without saying that we are in urgent need of a vaccine. Unless governments opt for the herd immunity option (which I sure hope they don’t), a vaccine will be the only means of controlling the pandemic, long-term. Even a moderately effective vaccine could drastically reduce the immense pressure on hospital intensive care units and supply chains, while allowing economies to begin their slow recovery.
The research race is now on. Over 80 vaccine programs have been announced by an amalgamation of academic, biopharmaceutical, governmental and non-governmental organisations [4], comprising a diverse range of technologies and platforms. In the following sections, we discuss the major strategies for COVID-19 vaccine development and outline the challenges involved.
Subunits and Peptides
Subunits are a textbook vaccine strategy, in which pathogen-derived antigenic fragments (most commonly proteins) are used to stimulate an immune response. For SARS-CoV-2, Spike glycoprotein is the subunit of choice. Naturally found as a homotrimer, Spike studs the viral envelope and gives the coronaviruses their characteristic crown-like appearance. On a structural level, Spike’s ectodomain can be divided into two functionally-distinct subunits:
+ Subunit 1 (S1), which mediates attachment and binding of SARS-CoV-2 virions to human ACE2 receptors via its receptor-binding domain (RBD) [5].
+ Subunit 2 (S2), which undergoes dramatic structural changes to fuse with host or viral membranes and mediate entry [6].
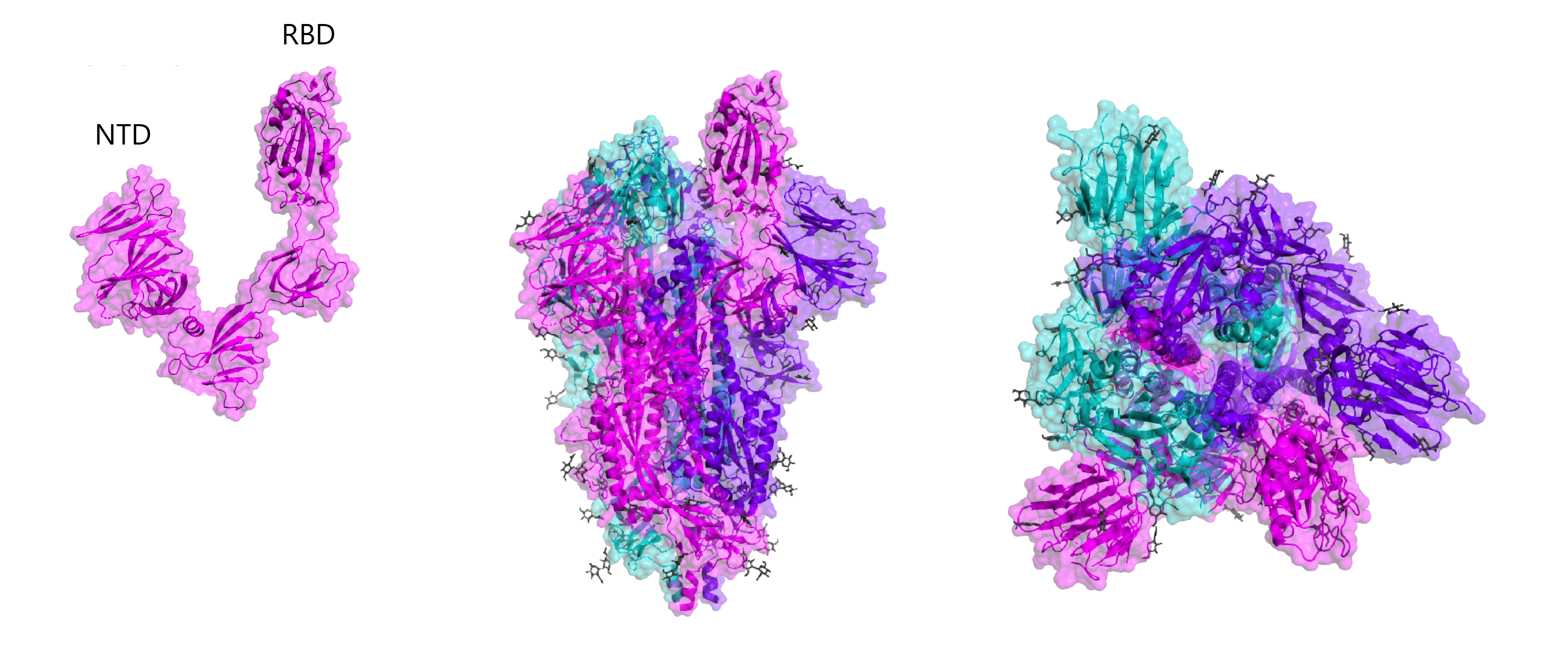
X-ray crystal structures of the SARS-CoV-2 Spike protein. Left: Spike subunit 1 (S1), with RBD and N-terminal domain labelled. Middle: Side-on view of Spike trimer in open conformation. Right: Top-down view of Spike trimer in open conformation. Glycans are shown grey. Structure determined by Walls and colleagues (RCSB: 6VYB).
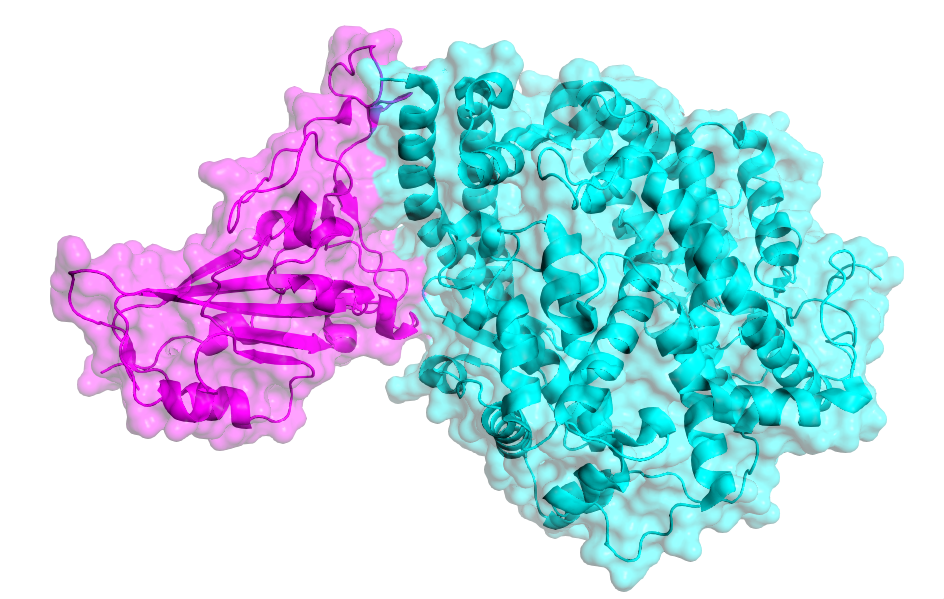
SARS-CoV-2 Spike RBD (magenta) bound to human ACE2 (cyan). Structure determined by Lan and colleagues (RCSB: 6M0J).
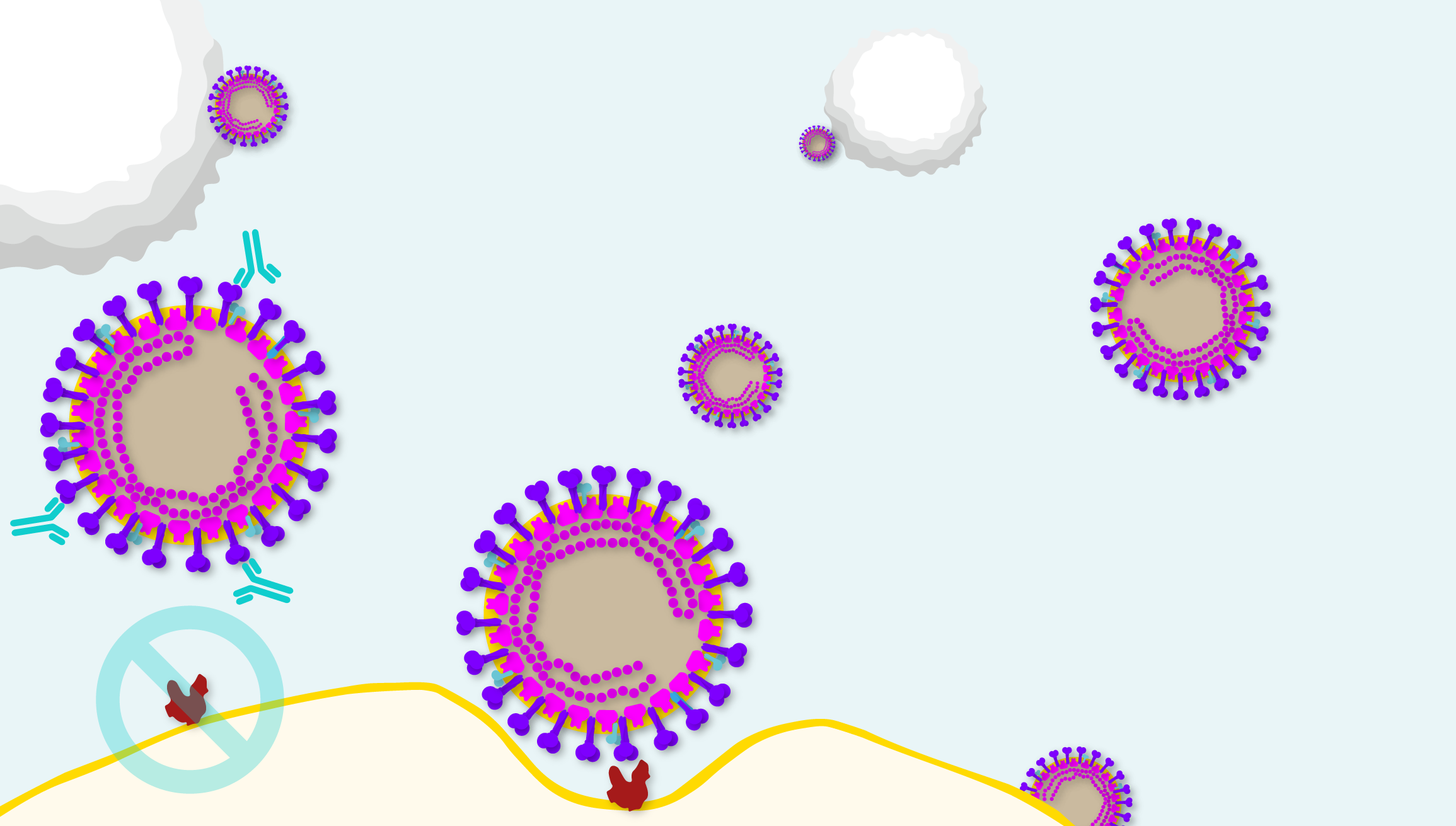
Diagram illustrating the point at which neutralising antibodies can block coronavirus pathogenesis.
The coronavirus Spike protein is a remarkable feat of viral evolution, having developed an elaborate mechanism to bind ACE2 and reconfigure it as an entry point to host cells.
Yet, as Spike must protrude from the viral capsid to effectively bind ACE2, it also draws the ire of the immune response. Indeed, Spike proteins induce the highest neutralising antibody (nAb) titres of any coronavirus antigens [7], making them prime candidates for vaccine development, as they could preclude SARS-CoV-2-ACE2 interaction entirely to halt COVID-19 in its tracks.
But vaccine development is a complex and convoluted process. As subunits only comprise part of a virus, they can struggle to elicit a robust-enough immune response to confer adequate protection. Most Spike vaccines that were developed following the SARS and MERS outbreaks, for example, were only partially protective against viral challenge in animal models and failed to mimic the kind of immune responses observed with natural infections [9]. The reasons for these shortcomings are complex, but generally speaking, subunit vaccines have a poor track record of stimulating long-term humoral immunity and have a tendency to neglect cell-mediated responses, which are essential for clearing viral infection [10].
Nonetheless, nearly all subunit vaccine candidates for COVID-19 are based on Spike, with an ongoing debate on how to best improve its immunogenicity. Researchers have so-far used whole Spike or select regions of the protein, such as the RBD or N-terminal domain in combination with established or new adjuvants for an extra immunological kick. Researchers are also investigating the use of additional viral antigens, such as Nucleoprotein or those that are non-structural to create ‘cocktail’ vaccines [9]. Innovax, for example, are planning to screen a series of truncated Spike proteins in pre-clinical testing to select a lead candidate from the resulting immunogenicity data [11]. Their vaccine (XWG-03) will also be tested with an oil-in-water adjuvant (AS03) provided by GSK [12, 13].
Taking truncation one step further, it may be possible to elicit immune responses with shorter, antigenic peptides. Produced via chemical synthesis, peptide vaccines allow for greater scalability in manufacturing and quality control. However, their low molecular weight and structural variance has tended to result in low humoral immunogenicity, requiring the addition of various structural modifications and adjuvants that complicate vaccine design. US biotech, EpiVax are developing a cocktail vaccine of 20 peptides that aims to stimulate the SARS-CoV-2 T-cell response [14]. The intention is not to provide total protection, but to confer a degree of protection to healthcare workers as a ‘stop-gap’ until more protective vaccines becomes available.
Messenger RNA
Messenger RNA (mRNA) is a type of nucleic acid that, as the name suggests, acts as an intermediary ‘messenger’ between protein-coding DNA in the nucleus and translation in the cytoplasm. Unlike protein-based vaccines, mRNA vaccines are delivered directly into cells to produce antigens ‘on-site’. Once translated, these antigens undergo extracellular export for antibody recognition, or intracellular processing and presentation to T-cells, to build the humoral and cell-mediated immune responses, respectively. The idea being, that by producing antigens in vivo, a more ‘real’ immune response can be stimulated.
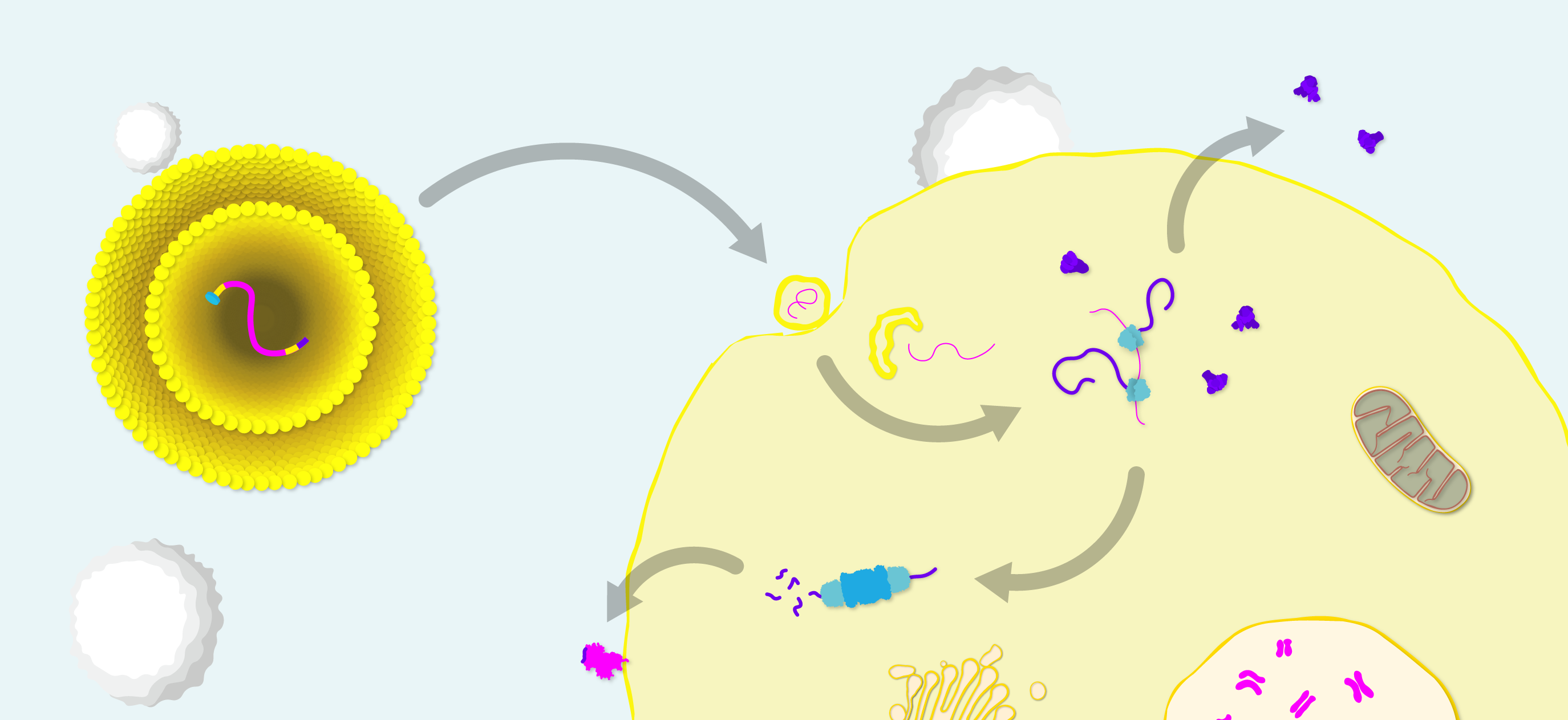
Diagram of mRNA vaccine structure and mode of action. Lipid nanoparticle (LNP)-formulated mRNA with antigen open reading frame (magenta), flanked by short untranslated regions (yellow), a 5’ m7G cap to initiate translation (cyan) and a 3’ polyA tail (purple) to protect the transcript from degradation. Upon entry to the cell, mRNA is released from the LNP and translated by ribosomes into Spike protein. Spike is then exported for antibody recognition and processed by the immunoproteosome for T-cell recognition.
Because mRNA vaccines express antigens in vivo, proper protein folding, post-translational modifications and antigen localisation can be achieved – theoretically allowing mRNA vaccines to stimulate more ‘on-target’, potent immune responses. Another advantage is design flexibility, as the small size of mRNA molecules allows multiple sequences to be selected, edited and combined in a single formulation to deliver a broader antigenic payload or more complex, multimeric antigens. Sequences can also be further modified to recruit cellular machinery for transcript amplification to extend the length of the immune response [15].
Exogenous mRNA also has some unique immunological features. It its naked form, mRNA is inherently immunostimulatory and can act as its own adjuvant by activating various cell-surface, endosomal and cytosolic immune receptors [16]. However, the immunogenicity of mRNA can be a double-edged sword. For example, systemic type I IFN responses can inhibit translation factors that grind cellular protein production to a halt and lead to unwarranted side effects [17].
A major challenge for these vaccines is the inherent instability of single-stranded mRNA, and the added difficultly of intracellular delivery, which both require the development of additional technologies for storage and administration.
Regardless, mRNA vaccines show promise as a game-changing technology, and are so far leading the COVID-19 race. mRNA vaccine pioneer, Moderna Therapeutics have already announced the start of Phase I trials for their vaccine, mRNA-1273, which comprises an LNP containing mRNA that encodes the prefusion-stabilised form of Spike [18]. Given that no mRNA vaccine has ever gained regulatory approval, researchers are still eagerly awaiting a proof-of-concept approval. Earlier this year, Moderna’s mRNA vaccine for CMV was the first of its kind to make it to Phase III trials, and will be an important indicator of the platform’s success.
What About DNA?
Similar to mRNA, DNA vaccines are a nucleic acid platform (in this case, dsDNA plasmids), based on intracellular administration to express antigens in vivo. However, unlike mRNA, DNA is a highly stable molecule and can induce long-lasting antigen expression to stimulate a more robust immune response.
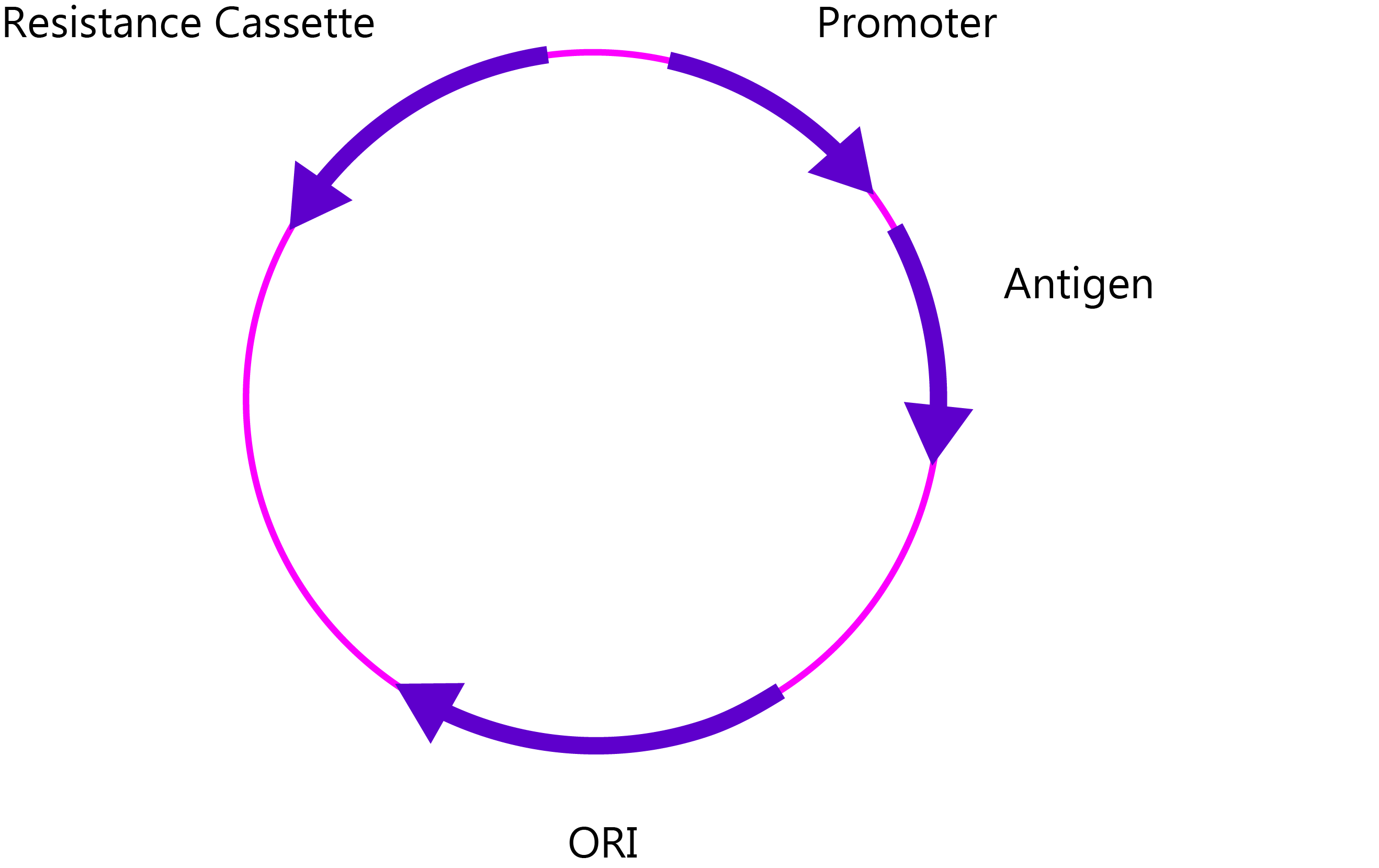
DNA vaccine plasmid, including antibiotic resistance cassette for selection in bacterial culture, promoter, antigen and origin of replication (ORI).
However, the caveat of DNA vaccines is the risk of host genome integration from extrachromosomal DNA in the nucleus via homologous recombination. What’s more, when you consider that plasmids can persist for a long time after injection (nearly 2 years by one account [19]), the risks of oncogenic mutation can give regulators plenty of cause for concern.
Unperturbed, Inovio Pharmaceuticals (USA) are developing a candidate (INO-4800), which was purportedly designed in only 3 hours [20] and began human testing last week [21]. INO-4800 encodes the SARS-CoV-2 Spike protein and will be administered using Inovio’s proprietary electroporation technology [22]. While it’s too early to speculate on INO-4800’s prospects, Inovio’s partner, GeneOne Life Sciences (South Korea), published promising Phase I data for their joint MERS DNA candidate (GLS-5300), showing good toleration and high levels of antibody titres in 95% of subjects, in addition to generating broad T-cell responses [23].
Attenuated and Inactivated Viruses
Whole virus vaccines are old-school strategy that date all the way back to vaccine pioneers, Edward Jenner and Louis Pasteur [24]. Attenuated whole virus vaccines are live viruses that are passaged in animals or tissue culture, so that they lose their virulence, whereas whole virus inactivation is achieved by various physical and chemical methods to render the virus ‘dead’, yet still immunogenic.
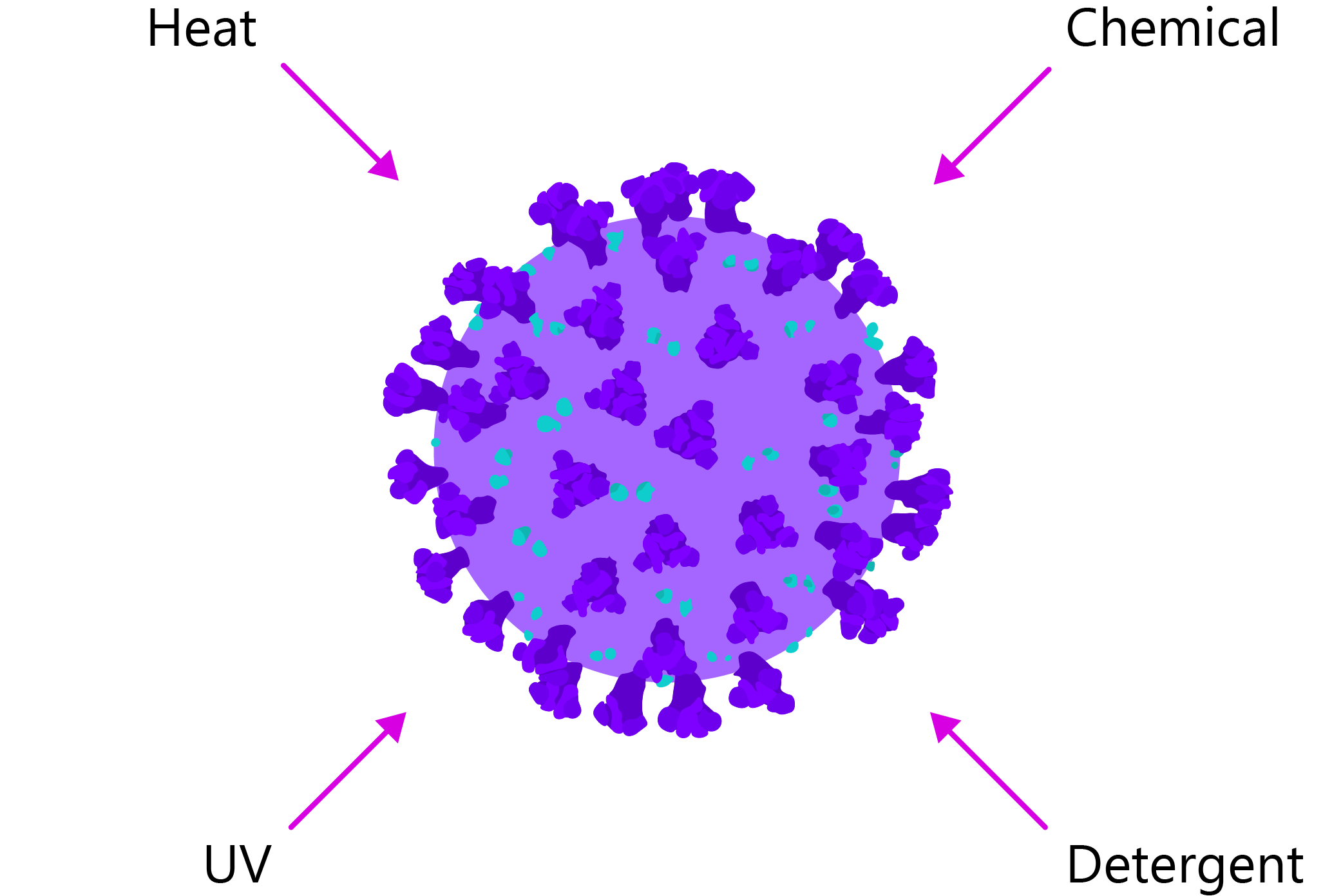
Diagram illustrating various methods that can be used to inactivate live virus.
The obvious advantage of whole vaccines is that they include all of a virus’s natural components, including its proteins, lipids, glycans and nucleic acids, facilitating broad and potent immune responses that tend to outperform other platforms. Live attenuated vaccines (LAVs) also have the added advantage (or disadvantage, depending how you look at it) of being able to self-replicate to further prolong the immune response.
This breadth of immunogenicity will be a crucial advantage in the timely development of a vaccine, as rational design strategies, such as subunits or nucleic acids can put the cart before the horse by defining the antigenic target prior to understanding how protective immunity is conferred.
So what’s the catch? Well, developing live viruses for attenuation and inactivation is a hazardous process, with stringent quality control implications and plenty of hurdles in the way of reaching regulatory approval. Attenuated vaccines also risk gain-of-function mutations or recombination with other co-infecting viruses, that cause reversion to a virulent strain [25]. Furthermore, while whole virus formulations may be non-infectious, that is not to say that they don’t induce harmful immune responses. This is where the ‘whole-ness’ of attenuated and inactivated vaccines is less desirable, as they cannot be as easily customised like subunit or nucleic acid methods.
A couple of inactivated and attenuated SARS vaccines have showed protection against live SARS challenge in mouse models and the reduction in viral titres [26, 27], though the body of research in this area is far from comprehensive. Chinese company, Sinovac Biotech, recently received approval to test their inactivated SARS-CoV-2 vaccine in human clinical trials and have teamed up with Dynavax for an adjuvant [28]. While there is little information yet available on their candidate, or mode of inactivation, Sinovac have seen success in the rapid development and approval of pandemic influenza vaccines.
Virus-Like Particles
Virus-like particles (VLPs) comprise the self-assembling structural proteins of a virus but lack its genome. These viral ‘shells’ therefore combine the best traits of subunit and whole virus vaccines, as they are large and non-infectious, yet antigenically heterogeneous.
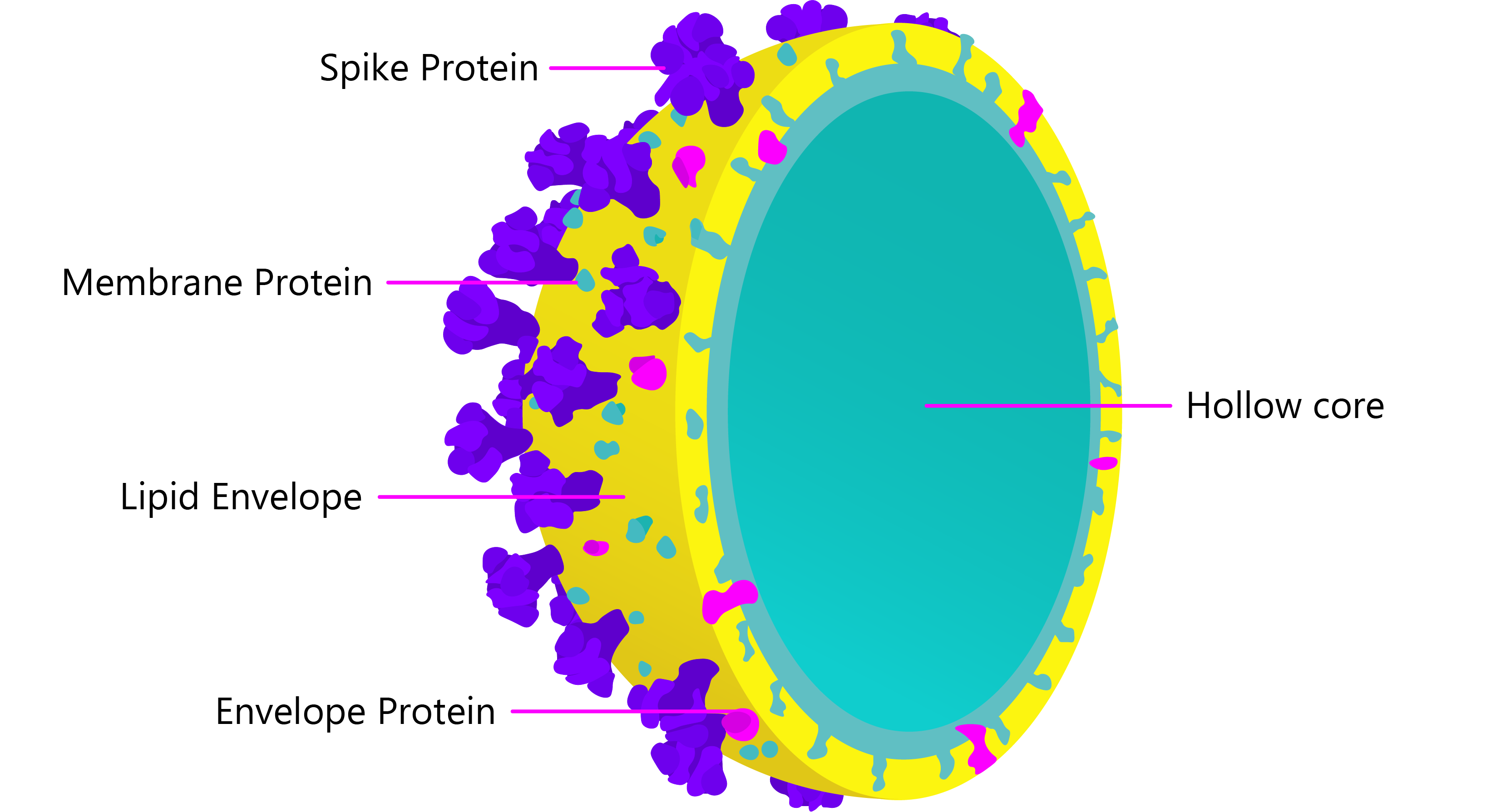
Cross-section of non-enveloped SARS-CoV-2 virus-like particle, comprised of self-assembling Membrane and Spike glycoproteins.
As VLPs structurally mimic infectious viruses, they are able to induce potent humoral and cellular immune responses without the use of adjuvants [29]. The large, rigid and repetitive surface epitopes of VLPs create more effective pathogen-associated molecular patterns (PAMPs) than soluble proteins, and as many components of the innate humoral response are multimeric (IgM, complement), their binding avidity is greatly improved to facilitate cross-linking, opsonisation and antigen uptake.
The particulate nature of VLPs also allows them to induce potent T-cell mediated immune responses via interaction with antigen-presenting cells (APCs) – especially dendritic cells [30]. Due to the high surface density of PAMPs, uptake of a single VLP by an APC can feed thousands of epitopes into the processing and presentation machinery, further enhancing their potency and induction of CD8+ T-cells [30].
While no coronavirus vaccines based on VLPs have made it out of pre-clinical testing [31, 32], the few studies available have showed good antibody and T-helper cell responses in animal models [33, 34], and have even shown to protect mice from lethal challenge [35]. Canadian biotech, Medicago have announced the development of a SARS-CoV-2 VLP, grown in their tobacco plant expression system, which is highly scalable for protein production [36]. However, as plants lack the same glycosylation machinery of mammalian cells, they are unable to produce fully post-translationally modified proteins and so may not elicit the specificity of immune responses required [37].
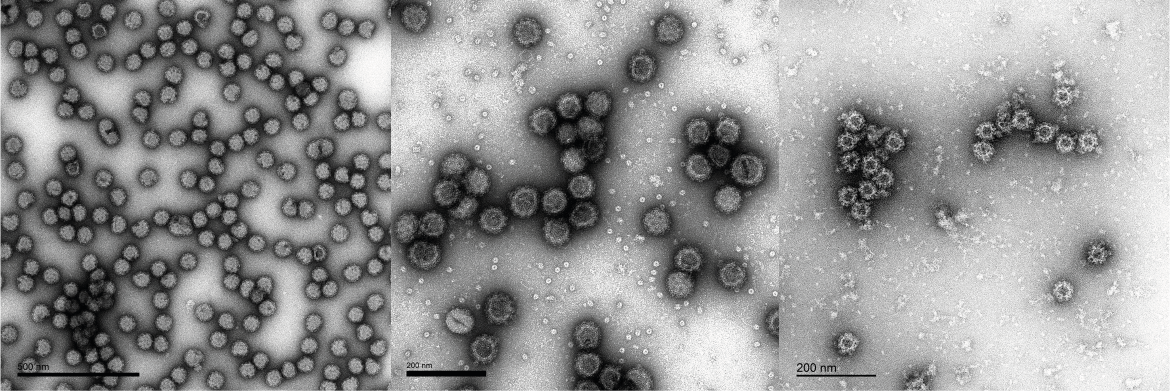
Transmission electron microscope (TEM) images of Mayaro, Noro- and O’nyong’nyong virus-like particles produced by The Native Antigen Company.
Viral Vectors
Vectored vaccines are non-pathogenic viruses that have been engineered to carry the genetic material of another virus and deliver it into cells for antigen production, in vivo. The most commonly-used vectors are the adeno-, measles and modified Vaccinia Ankara viruses (MVA).
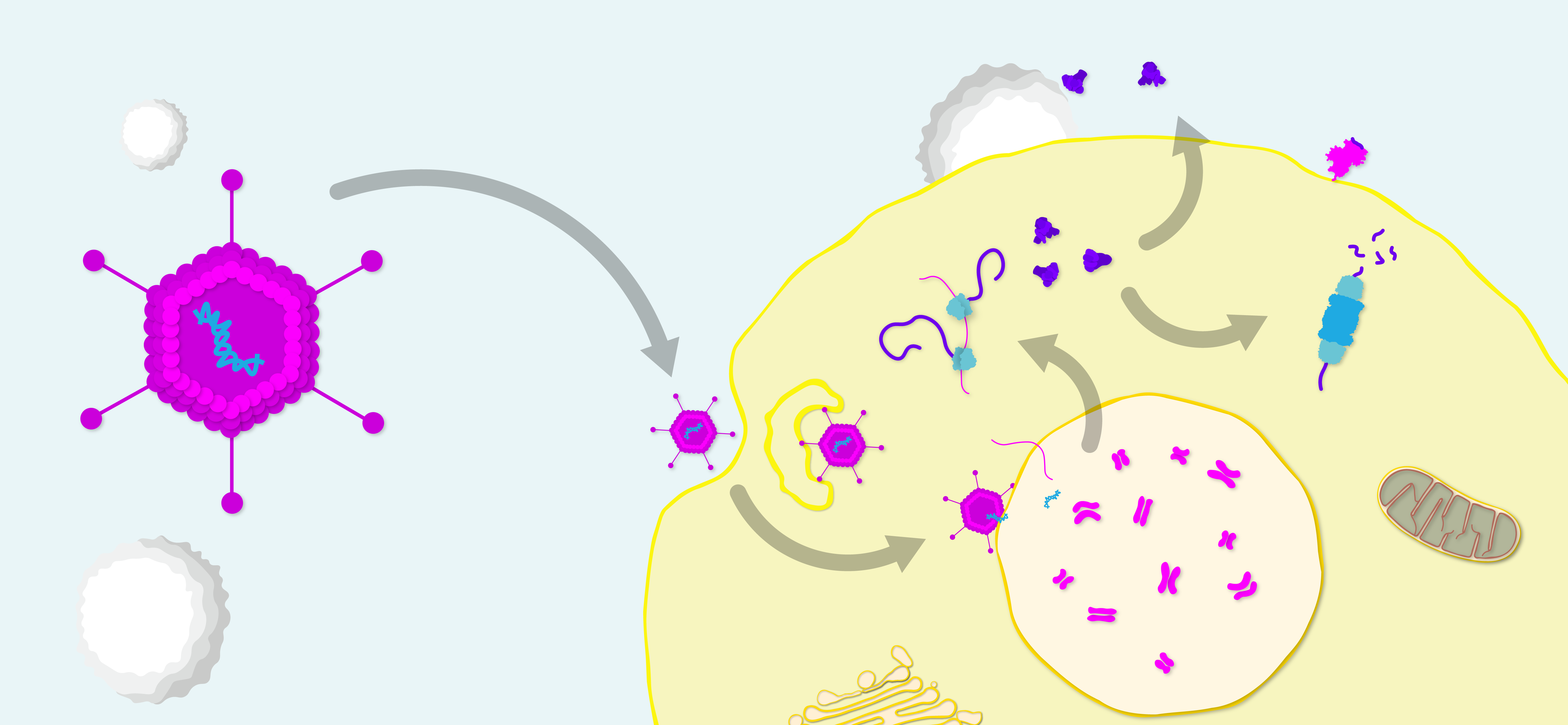
Diagram of adenovirus vector vaccine generating humoral and cell-mediated immune responses. The adenovirus enters the host cell and releases its DNA into the nucleus. The DNA is transcribed into RNA, which is exported to the cytoplasm and translated into antigen. Antigens are then excreted for humoral recognition and processed for presentation to T-cells.
Like nucleic acid vaccines, viral vectors are ‘platform’ technologies that can be modified to express any antigen(s) of choice, and deliver the target genome for transcription/translation in vivo to facilitate native expression and post-translational modification of antigens.
As vectored vaccines deliver antigens intracellularly, they can induce strong T-cell responses and don’t need to rely on adjuvants. MVA and parainfluenza vectors expressing SARS-CoV Spike were also shown to elicit neutralising antibodies that protected mice from immune sera and SARS challenge, respectively [38, 39].
However, as genetically-modified organisms (GMOs), vaccine vectors are subject to stringent risk assessment – less than ideal in a pandemic scenario. Safety concerns also arise from potential integration of DNA with host genome, or persistent viral replication if attenuation is insufficient. Also, if a vaccine recipient has already developed immunity to the vector in question, then the recipient’s immune response will severely limit transduction efficiency and expression of the transgene(s) [40]. To counter this, novel vectors are often used, to which humans are immunologically naïve.
The University of Oxford (UK) have opted for their chimpanzee adenovirus vaccine vector (ChAdOx1 nCoV-19), encoding the SARS-CoV-2 Spike protein, which is now being testing in Phase I trials. ChAdOx1 has shown efficacy in various clinical trials, including those for MERS, Chikungunya and Zika, and is also being evaluated as a cancer vaccine platform [41].
The Vaccine Landscape
We may well be in the midst of one of the biggest medical research races in history. Since its genome was sequenced in early January, research on SARS-CoV-2 has progressed at record-breaking speeds, with a multitude of different vaccine technologies now in the pipeline.
Considering that no coronavirus vaccine has ever progressed past Phase I trials and that many of the platforms in development have never seen regulatory approval, a vaccine in 12-18 months will not be an easy feat, as development and testing typically takes years, if not decades, with a high rate of attrition for even the most promising candidates. Nonetheless, the global vaccine response has so far been promising, and will likely yield some exciting breakthroughs in the coming months. While every vaccine is a long shot, some are just a bit longer than others.
WRAIR Sanofi Pasteur |
|||
Axon Neuroscience |
|||
CureVac Imperial College London |
|||
Karonlinska Institute Applied DNA Sciences |
|||
Wuhan Institute of Virology BIKEN |
|||
Expression Biotechnologies Imophoron |
|||
CanSino Biological GeoVax |
Supporting Vaccine R&D at The Native Antigen Company
In February 2020, The Native Antigen Company became one of the world’s first suppliers to release commercially-available SARS-CoV-2 antigens. Since then, our scientists have been hard at work, extending our coronavirus range to support the research and development of urgently-needed diagnostics and vaccines. Our antigens are produced using our proprietary, VirtuE (HEK293) expression system, which ensures full glycosylation and proper folding, to ensure full biological and antigenic activity.
You can keep up-to-date with new product developments on our Coronavirus Pipeline page. The Native Antigen Company offers an extensive and growing range of reagents for the human coronaviruses, including the emergent SARS-2, SARS, MERS coronaviruses, as well as the OC43, HKU1, 229E, and NL63 endemic coronaviruses.
If we are not currently developing something that you’re interested in, please get in touch at contact@thenativeantigencompany.com, as we may be able to develop it for you.
SARS-CoV-2 Spike Glycoprotein (S2) (aa 1000-1200), His-Tag (E. coli)
$640.54 – $2,403.48 excl. VATSARS-CoV-2 Papain-Like Protease (PLpro), Active
$928.08 – $1,324.69 excl. VATMERS Coronavirus Spike Glycoprotein (S1), Camel Fc-Tag (HEK293)
$749.60 excl. VATSARS-CoV-2 Nucleoprotein, His-Tag (E. coli)
$658.38 – $2,508.58 excl. VATHuman Coronavirus HKU1 Nucleoprotein, His-Tag (E. coli)
$640.54 – $2,403.48 excl. VATHuman Coronavirus HKU1 Spike Glycoprotein (Full-Length), Sheep Fc-Tag (HEK293)
$943.93 – $3,585.38 excl. VATHuman Coronavirus OC43 Nucleoprotein, His-Tag (E. coli)
$640.54 – $2,403.48 excl. VATSARS-CoV-2 Spike Glycoprotein (S1) RBD, His-Tag (HEK293)
$652.44 – $2,486.76 excl. VATSARS-CoV-2 Spike Glycoprotein (S1), His-Tag (Insect Cells)
$715.90 – $2,720.76 excl. VATHuman Coronavirus 229E Spike Glycoprotein (S1), Sheep Fc-Tag (HEK293)
$749.60 – $2,817.95 excl. VATHuman Coronavirus 229E Nucleoprotein, His-Tag (E. coli)
$640.54 – $2,403.48 excl. VATSARS-CoV-2 Spike Glycoprotein (S2), His-Tag (Insect Cells)
$715.90 – $2,720.76 excl. VATSARS-CoV-2 Spike Glycoprotein (Full-Length), His-Tag (CHO)
$989.55 – $3,763.85 excl. VATSARS Coronavirus Nucleoprotein (N-Term) (E. coli)
$640.54 – $2,403.48 excl. VATSARS Coronavirus Nucleoprotein (C-Term) (E. coli)
$640.54 – $2,403.48 excl. VATSARS Coronavirus Membrane Protein (Matrix) (E. coli)
$640.54 – $2,403.48 excl. VATSARS Coronavirus Envelope Protein (E. coli)
$640.54 – $2,403.48 excl. VATSARS-CoV-2 Spike N-Terminal Domain (NTD), Sheep Fc-Tag (HEK293)
$658.38 – $2,508.58 excl. VATSARS-CoV-2 Spike Glycoprotein (Trimeric), His-Tag (CHO)
$1,035.15 – $3,934.40 excl. VATSARS-CoV-2 Spike Glycoprotein (S2) (aa 800-1000), His-Tag (E. coli)
$640.54 – $2,403.48 excl. VATHuman Coronavirus OC43 Spike Glycoprotein (Full-Length), Sheep Fc-Tag (HEK293)
$943.93 – $3,585.38 excl. VATSARS-CoV-2 Spike Glycoprotein (S1), Sheep Fc-Tag (CHO)
$771.42 – $2,930.97 excl. VATSARS-CoV-2 Spike Glycoprotein (S2), Sheep Fc-Tag (CHO)
$771.42 – $2,930.97 excl. VATSARS-CoV-2 Spike-E-M Mosaic Protein, His-Tag (E. coli)
$658.38 – $2,508.58 excl. VATHuman Coronavirus OC43 Purified Viral Lysate
$797.20 – $3,391.04 excl. VATHuman Coronavirus NL63 Purified Viral Lysate
$797.20 – $3,391.04 excl. VATHuman Coronavirus 229E Purified Viral Lysate
$797.20 – $3,391.04 excl. VATHuman ACE2 (18-615) Recombinant Protein, Sheep Fc-Tag (HEK293)
$737.69 – $2,800.09 excl. VATSARS-CoV-2 Spike Glycoprotein (S1), Sheep Fc-Tag (HEK293)
$771.42 – $3,519.94 excl. VATSARS-CoV-2 Spike Glycoprotein (S2), Sheep Fc-Tag (HEK293)
$771.42 – $2,930.97 excl. VATSARS Coronavirus Nucleoprotein, His-Tag (HEK293)
$654.41 – $2,494.69 excl. VATSARS Coronavirus Spike Glycoprotein (S1), His-Tag (HEK293)
$749.60 – $2,855.61 excl. VATSARS-CoV-2 RNA-Dependent RNA Polymerase (RdRp), Active
$967.73 – $3,825.34 excl. VATSARS-CoV-2 3C-Like Proteinase (3CLpro), Active
$906.27 – $1,279.09 excl. VATHuman Coronavirus NL63 Spike Glycoprotein (Full-Length), Sheep Fc-Tag (HEK293)
$943.93 – $3,585.38 excl. VATHuman Coronavirus 229E Spike Glycoprotein (Full-Length), Sheep Fc-Tag (HEK293)
$943.93 – $3,585.38 excl. VATHuman Coronavirus HKU1 Spike Glycoprotein (S1), His-Tag (HEK293)
$749.60 – $2,817.95 excl. VATHuman Coronavirus 229E Spike Glycoprotein (S1), His-Tag (HEK293)
$749.60 – $2,817.95 excl. VATHuman Coronavirus NL63 Spike Glycoprotein (S1), His-Tag (HEK293)
$749.60 – $2,817.95 excl. VATHuman Coronavirus OC43 Spike Glycoprotein (S1), His-Tag (HEK293)
$749.60 – $2,817.95 excl. VATMERS Coronavirus Spike Glycoprotein (S1), His-Tag (Insect Cells)
$694.07 excl. VATSARS-CoV-2 Spike N-Terminal Domain (NTD), His-Tag (HEK293)
$658.38 – $2,508.58 excl. VATSARS-CoV-2 Stabilized Spike Glycoprotein (Full-Length), His-Strep-Tag (HEK293)
$989.55 – $3,763.85 excl. VATSARS-CoV-2 NSP7 Protein, His-Tag (E. coli)
$501.72 – $961.79 excl. VAT
Rabbit IgG Anti SARS-CoV-2 Nucleoprotein Polyclonal Antibody
$630.62 – $1,957.29 excl. VATRabbit IgG Anti SARS-CoV-2 Spike (S1) RBD Polyclonal Antibody
$630.62 – $1,957.29 excl. VATRabbit IgG Anti-SARS-CoV-2 Spike (S2) Polyclonal Antibody
$630.62 – $1,957.29 excl. VATRabbit IgG Anti-SARS-CoV-2 Spike (S1) Polyclonal Antibody
$630.62 – $1,957.29 excl. VATMouse Anti SARS-CoV-2 Spike (S1) Antibody (EB5)
$696.06 – $2,090.14 excl. VATMouse Anti SARS-CoV-2 Spike (S1) Antibody (FH4)
$696.06 – $2,090.14 excl. VATHuman IgM Anti-SARS-CoV-2 Spike (S1) Antibody (CR3022)
$1,674.59 excl. VATMouse Anti SARS-CoV-2 Spike (S1) Antibody (HH10)
$696.06 – $2,090.14 excl. VATRabbit IgG Anti-SARS-CoV-2 Spike (S1) Antibody (CR3022)
$761.50 – $2,899.24 excl. VATMouse Anti SARS-CoV-2 Spike (S1) RBD Antibody (CE5)
$696.06 – $2,090.14 excl. VATMouse Anti-SARS Coronavirus Nucleoprotein Antibody (3862)
$428.34 – $1,074.82 excl. VATMouse Anti SARS-CoV-2 Spike (S1) RBD Antibody (DH6)
$696.06 – $2,090.14 excl. VATMouse Anti-SARS Coronavirus Nucleoprotein Antibody (3863)
$428.34 – $1,074.82 excl. VATMouse Anti SARS-CoV-2 Spike (S1) RBD Antibody (FG11)
$696.06 – $2,090.14 excl. VATMouse Anti-SARS Coronavirus Nucleoprotein Antibody (3864)
$428.34 – $1,074.82 excl. VATMouse Anti SARS-CoV-2 Spike (S2) Antibody (AD10)
$696.06 – $2,090.14 excl. VATMouse Anti SARS-CoV-2 Spike (S2) Antibody (CH1)
$696.06 – $2,090.14 excl. VATMouse Anti SARS-CoV-2 Spike (S2) Antibody (HA11)
$696.06 – $2,090.14 excl. VATMouse Anti SARS-CoV-2 Spike (S2) Antibody (JC7)
$696.06 – $2,090.14 excl. VATMouse Anti-SARS Coronavirus Nucleoprotein Antibody (3851)
$428.34 – $1,386.07 excl. VATMouse Anti-SARS Coronavirus Nucleoprotein Antibody (3861)
$428.34 – $1,386.07 excl. VATMouse Anti-MERS Coronavirus Spike (S1) Antibody (3871)
$428.34 – $1,074.82 excl. VATMouse Anti-MERS Coronavirus Spike (S1) Antibody (3872)
$428.34 – $1,074.82 excl. VATMouse Anti-MERS Coronavirus Spike (S1) Antibody (3873)
$428.34 – $1,074.82 excl. VATHuman IgA Anti-SARS-CoV-2 Spike Antibody (A60H)
$466.02 – $1,219.59 excl. VATHuman IgG1 Anti-SARS-CoV-2 Nucleoprotein Antibody (CR3009)
$863.74 – $3,670.87 excl. VATRabbit IgG Anti-SARS-CoV-2 Nucleoprotein Antibody (CR3018)
$761.50 – $2,899.24 excl. VATHuman IgG1 Anti-SARS-CoV-2 Nucleoprotein Antibody (CR3018)
$761.50 – $2,899.24 excl. VATRabbit IgG Anti-SARS-CoV-2 Nucleoprotein Antibody (CR3009)
$761.50 – $2,899.24 excl. VATHuman IgM Anti-SARS-CoV-2 Nucleoprotein Antibody (CR3018)
$1,674.59 excl. VATHuman IgM Anti-SARS-CoV-2 Nucleoprotein Antibody (CR3009)
$1,674.59 excl. VATHuman IgG1 Anti-SARS-CoV-2 Spike NTD Antibody, Chimeric mAb (AM121)
$1,300.88 – $5,526.80 excl. VATRabbit IgG Anti HCoV-229E Spike (S1) Polyclonal Antibody
$630.62 – $1,957.29 excl. VATRabbit IgG Anti HCoV-OC43 Spike (S1) Polyclonal Antibody
$630.62 – $1,957.29 excl. VATRabbit IgG Anti HCoV-NL63 Spike (S1) Polyclonal Antibody
$630.62 – $1,957.29 excl. VATRabbit IgG Anti HCoV-HKU1 Spike (S1) Polyclonal Antibody
$630.62 – $1,957.29 excl. VATMouse Anti SARS-CoV-2 Nucleoprotein Antibody (GC12)
$434.29 – $1,080.78 excl. VATMouse Anti SARS-CoV-2 Nucleoprotein Antibody (JB8)
$434.29 – $1,080.78 excl. VATMouse Anti SARS-CoV-2 Nucleoprotein Antibody (JA6)
$434.29 – $1,080.78 excl. VATMouse Anti SARS-CoV-2 Nucleoprotein Antibody (JF1)
$434.29 – $1,080.78 excl. VATHuman IgG1 Anti SARS-CoV-2 Spike (S1) Antibody (EB5)
$583.02 – $2,040.58 excl. VATHuman IgG1 Anti SARS-CoV-2 Spike (S1) Antibody (HH10)
$583.02 – $2,040.58 excl. VATHuman IgG1 Anti SARS-CoV-2 Spike (S1) RBD Antibody (CE5)
$583.02 – $2,040.58 excl. VATHuman IgG1 Anti SARS-CoV-2 Spike (S2) Antibody (CH1)
$583.02 – $2,040.58 excl. VATHuman IgG1 Anti SARS-CoV-2 Spike (S1) RBD Antibody (DH6)
$583.02 – $2,040.58 excl. VAT
References
1. https://coronavirus.jhu.edu/map.html
3. https://www.newsweek.com/hydroxychloroquine-coronavirus-france-heart-cardiac-1496810
4. https://www.nature.com/articles/d41573-020-00073-5
5. https://jvi.asm.org/content/86/5/2856
6. https://www.biorxiv.org/content/10.1101/2020.02.19.956235v1
7. https://www.sciencedirect.com/science/article/pii/S0092867420302622
8. https://www.nature.com/articles/s41586-020-2180-5
9. https://www.biorxiv.org/content/10.1101/2020.03.20.000141v1
10. https://www.ncbi.nlm.nih.gov/pubmed/15962478
11. https://www.biospace.com/article/gsk-strikes-vaccine-deal-with-china-s-innovax-against-covid-19
13. https://www.tandfonline.com/doi/abs/10.1586/erv.11.192?journalCode=ierv20
14. https://epivax.com/pipeline/epv-cov19
15. https://www.ncbi.nlm.nih.gov/pmc/articles/PMC5906799/
16. https://www.nature.com/articles/nrd.2017.243
17. https://www.frontiersin.org/articles/10.3389/fimmu.2018.01963/full
19. https://link.springer.com/article/10.1385/MB:27:2:109
23. https://www.thelancet.com/journals/laninf/article/PIIS1473-3099(19)30266-X/fulltext
24. https://www.vbivaccines.com/wire/edward-jenner-and-the-first-modern-vaccine/
25. https://www.ncbi.nlm.nih.gov/pubmed/25864107
26. https://www.microbiologyresearch.org/content/journal/jgv/10.1099/vir.0.81579-0
27. https://jvi.asm.org/content/92/17/e00710-18
28. http://www.sinovac.com/?optionid=754&auto_id=896
29. https://pubmed.ncbi.nlm.nih.gov/30173619/
30. https://www.tandfonline.com/doi/full/10.4161/hv.22218
33. https://www.ncbi.nlm.nih.gov/pmc/articles/PMC5355045/
34. https://onlinelibrary.wiley.com/doi/full/10.1111/j.1365-2567.2010.03231.x
35. https://www.sciencedirect.com/science/article/pii/S0264410X1101005X?via%3Dihub
36. https://www.pmi.com/media-center/news/medicago-develops-a-plant-based-vaccine-for-coronavirus
37. https://www.ncbi.nlm.nih.gov/pmc/articles/PMC6097131/
38. https://www.ncbi.nlm.nih.gov/pmc/articles/PMC404098/
39. https://www.pnas.org/content/101/17/6641
40. https://www.ncbi.nlm.nih.gov/pmc/articles/PMC5721250/
41. https://www.vaccitech.co.uk/technology/